Abstract
Epigenetic mechanisms, primarily DNA methylation, histone and non-coding RNA modifications, play an important role in regulating gene expression. This review explores complex connections between epigenetics, evolution, viral infections, and cancer development. Specifically, it delves into the mechanisms induced by HPV and Influenza, the mechanisms that facilitate the development of Alzheimer’s disease, lung cancer and also the evolutionary origins and implications of epigenetics. Improving our understanding of these interactions on a molecular level can provide valuable insights for the development and advancement of diagnostic and therapeutic treatment, thereby improving clinical effectiveness.
1. Introduction
C. H. Waddington first introduced the term “epigenetics” in 1942 (Waddington C. H., 1942) to describe the study of the causal mechanisms by which the genes of a genotype lead to phenotypic effects. As of 2024, this branch of biology has grown considerably, with over 100,000 papers published on PubMed alone.
1.1 Mechanisms
The most well-studied mechanism of epigenetic modification is DNA cytosine methylation (Penner-Goeke S., and Binder E. B., 2024; De Lorenzi R., 2020), which is usually related to gene silencing (De Lorenzi R., 2020).
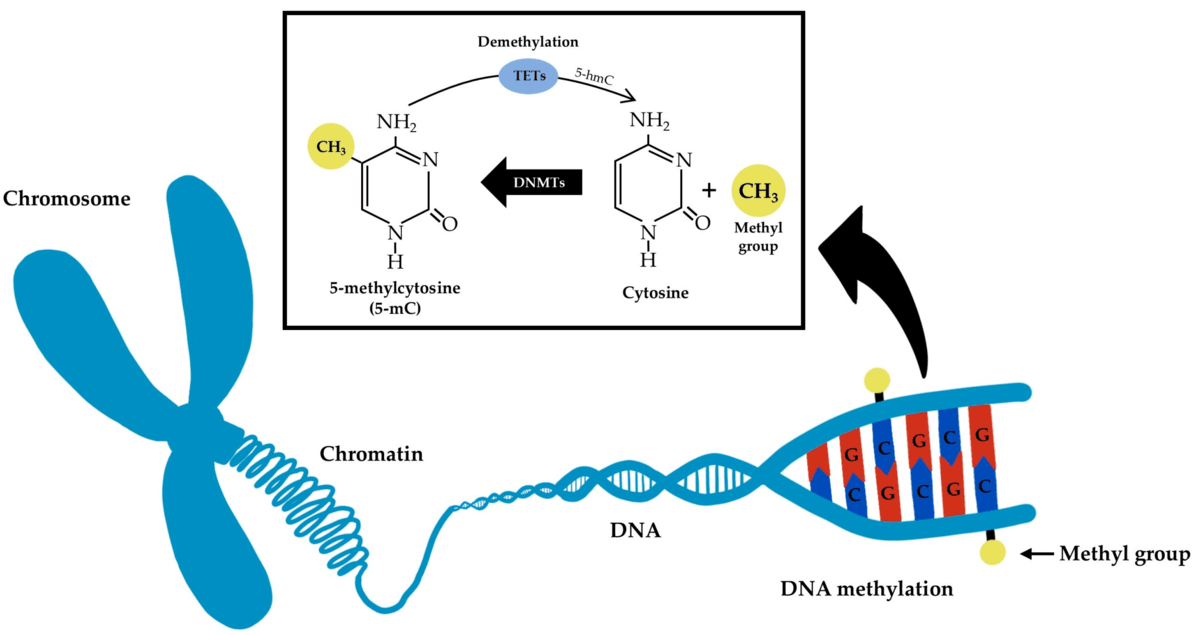
Figure 1: A simplified diagram of the process of DNA methylation and demethylation. Taken from https://www.mdpi.com/2079-4991/13/12/1880.
The DNA of eukaryotes and archaea is wrapped around structural proteins called histones, which are also targets of epigenetic modifications. Histone acetylation causes euchromatin formation due to the negatively charged acetyl groups, therefore binding less tightly to DNA, exposing the promoters of the associated genes.
Histone methylation is associated with both gene silencing and expression, depending on the position of the methyl group (Jackson R., et al, 2010). Repressive epigenetic marks include H2K4Me2, which means di-methylation in lysine residue of position 4, of H2 type histone.
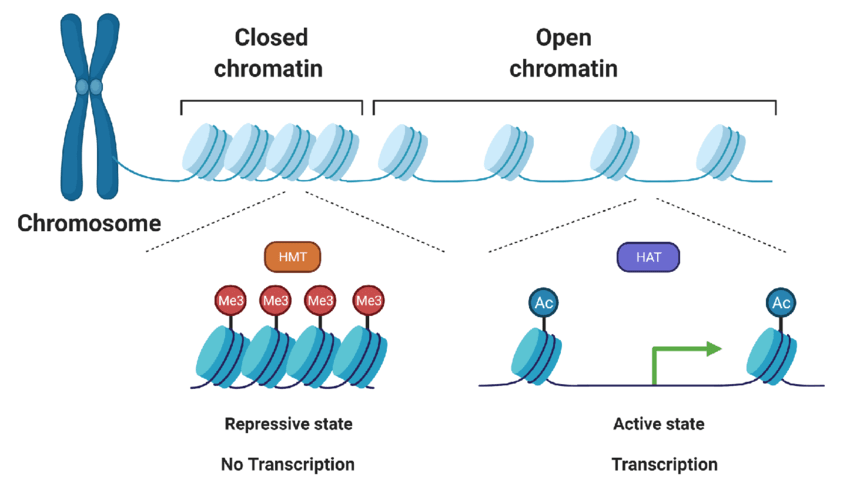
Figure 2: An overview of histone methylation (catalyzed by Histone methyltransferases, HMTs), Histone acetylation (catalyzed by Histone Acetyltransferases, HATs), and their effects on gene expression. Taken from https://www.researchgate.net/publication/364705421_Epigenetic_alterations_in_canine_mammary_cancer.
Other epigenetic modifications include histone phosphorylation, which can result in either gene silencing or activation, and SUMOylation, which refers to the addition of a small ubiquitin-like modifier (SUMO) to a lysine (K) residue on the histone.
1.2 Expanding Horizons: The Role of Epigenetics in Tumor-genesis, Autoimmune Disorders, Viral Infection, and treatment
Although it was first thought that the main role of epigenetic regulation was cell specialization (Bird A., 2007; Jaenisch R., and Bird A., 2003), research has shown that epigenetic regulation is involved in many more mechanisms (Rodger E.J., and Chatterjee A., 2017). These include mechanisms that contribute to tumor genesis (Lu Y., et al, 2020), autoimmune disorders such as Alzheimer’s disease (De Plano et al., 2024), and mechanisms that the host employs to counteract a viral attack (Roy A., and Ghosh A., 2024). Epigenetic regulation is also shown to be employed by some viruses themselves and used against the host (MacLennan S.A., and Marra M.A., 2023). This article explores the role of epigenetics in the regulation of viruses that integrate their genetic material into the host’s DNA, focusing on the example of HPV. We then move on to another example of a virus, Influenza. The focus here is on the use of epigenetic regulation by the virus against rather than by the host in order to control the virus. That way, another domain in which epigenetic research has been proven useful is introduced: the discovery of epidrugs. A range of well-studied epigenetic mechanisms that lead to malignancy are then explored. The purpose of this section is to underline another category of human disease in which epigenetics plays an important role. Falling into the autoimmune category (Weaver DF., 2023), the last example of human disease, in which epigenetic regulation shows great involvement, is Alzheimer’s. It is recognized as a huge public health challenge, already costing more than 1% of economic output based on medical care and projected to reach up to 2% by 2030 due to increasing prevalence. Alzheimer’s disease (AD) accounts for 60-70% of dementia cases, and results from a complex interplay of genetic, environmental, lifestyle, and aging factors. Epigenetic mechanisms act both in favor of and against the human organism, the last section is devoted to the evolutionary implications of the maintenance of epigenetic mechanisms, acting as a “balancer” of the proven “benefits and drawbacks” of an epigenetic modification machinery. An exploration of more of the evolutionary advantages of epigenetic mechanisms is made through the lenses of adaptation, survival, and speciation. The conclusions drawn by this section can be summarized in professor Carey’s quote “it would be a mistake to look back on Lamarck and only mock” (Mboma A., 2020).
1.3 Epigenetic inheritance
The increasing research on epigenetic mechanisms could be partly attributed to epigenetic inheritance, which has raised the question as to whether epigenetic modifications linked to diseases, such as Alzheimer’s, can be passed down through generations.
The two main types of epigenetic inheritance are Intergenerational and Transgenerational epigenetic inheritance (Fitz-James M.H., and Cavalli G., 2022). The former refers to the inheritance of epigenetic markers from parents to an F1 generation for males and F1 and F2 for females. The reason for this difference is explained in Figure 3. The latter refers to the transmission of epigenetic markers beyond the limits defying the intergenerational epigenetic inheritance. The existence of transgenerational epigenetic inheritance is harder to prove when it comes to the human species (Fitz-James M.H., and Cavalli G., 2022), due to other factors playing a role that cannot be easily controlled in studies, such as upbringing.
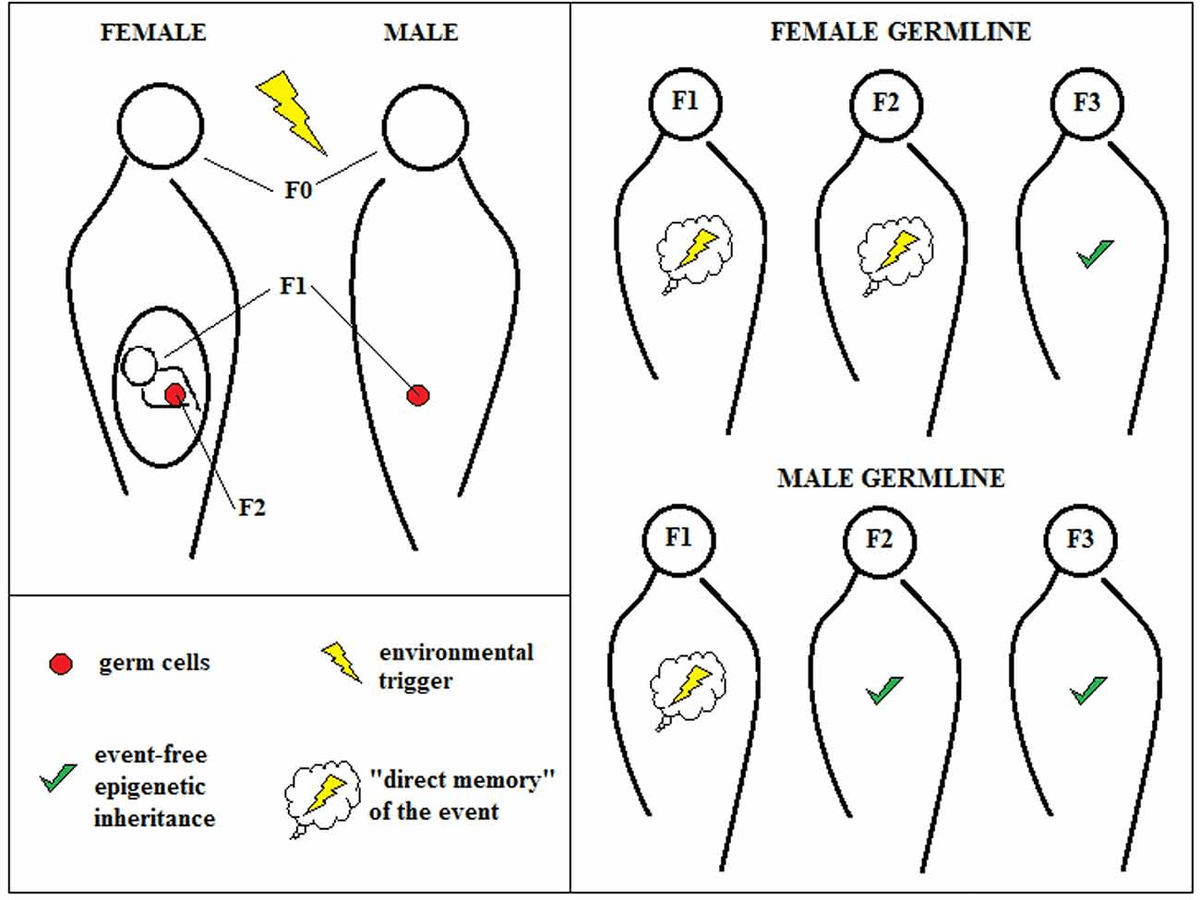
Figure 3: The enviornmental triggers that affect the F0 generation in females are thought to directly affect both the F1 and F2 generation, which explains the given definition for Transgenerational Epigenetic Inheritance. Taken from https://www.frontiersin.org/journals/molecular-neuroscience/articles/10.3389/fnmol.2018.00292/full.
2. Viruses: HPV
2.1 The HPV Virus
Human papillomaviruses (HPVs) are small, non-enveloped, double-stranded DNA viruses with approximately 8,000 base pairs in their genome (Mac and Moody, 2020; Burley et al., 2020). The DNA of HPV is associated with cellular histones, forming chromatin. These histones are subject to post-translational epigenetic modifications by the host cell and play an important role in regulating viral gene transcription (Burley et al., 2020). The epigenetic changes result in increased virus transcription and activation of the virus late promoter, facilitating transcription of the viral capsid proteins and the expression of key viral oncogenes including E6 and E7 (Burley et al, 2020; Ferguson et al., 2021). The main functions of E6 and E7 are to promote the degradation of the tumor suppressor protein p53 and disrupt the normal function of the retinoblastoma protein (pRb) respectively (Ye et al., 2023). Ultimately, the integration of the viral oncogenes E6 and E7 into the human host cell genome facilitates the development of HPV-associated cancers.
Throughout this tumorigenic process, post-translational modifications of histones, including acetylation, phosphorylation and methylation, as well as DNA methylation take place, altering the accessibility of chromatin (Mac and Moody, 2020). Since HPV comprises different viral variants, HPV 16 and HPV 18, which are responsible for the majority of HPV-related cancers, will mainly be discussed in the review.
2.2 Chromatin remodeling
Through epigenetic modifications, chromatin is remodeled into different physical states to regulate transcription. The two forms of chromatin are heterochromatin, a tightly-packed chromatin, and euchromatin, an open chromatin. Heterochromatin is transcriptionally repressed, whereas euchromatin is permissive for gene transcription as it contains far less densely packed histones, thus allowing transcription factors to drive transcription. These forms of chromatin are the results of the post-translational modification of histones or the methylation of DNA (Burley et al., 2020). Heterochromatin is formed due to the enrichment of repressive epigenetic marks such as H2K4Me2/3, H3K9Me1, H3K27Me2/3, and H4K20Me3 (Burley et al., 2020). In contrast, histone acetylation reduces the histones’ affinity for DNA, causing the chromatin to become more accessible. This open euchromatin is enriched with specific epigenetic marks, including the acetylation of H3K4 and the acetylation of H3K27 (Burley et al., 2020).
2.3 Histone acetylation
Histone acetylation is a reversible process mediated by histone acetyltransferase (HATs). Histones alter the spatial structure of nucleosomes and induce chromatin loosening through HATs. When it removes acetyl groups through histone deacetylases (HDACs), the chromatin structure is condensed, thereby regulating gene expression and influencing cervical cancer progression. In cervical cancer cells, Tip60, a transcription factor with HAT (histone acetyltransferase) activity, plays an inhibitory role in the development of the cancer, while P300 plays a facilitating role. Tip60 increases acetylation of histones H3K27 and H4K5 and represses HPV18 E6/E7 genes, whereas HAT p300 does the opposite, enhancing histone H3K27, H3K9 and H4K16 acetylation and activating the transcription of HPV18 E6/E7 (Lai et al., 2020). Since HPV18 LCR is a bivalent chromatin, transcription factors Tip60 and p300 are required to balance the active and repressive histone modifications. Therefore, the antagonistic roles of Tip60 and p300 make it a potential therapeutic target in cervical cancer (Lai et al., 2020). In addition, histone H3 and H4 acetylation are associated with the activation of HPV16 gene expression in cervical cancer cells and that the level of histone acetylation is positively correlated with HPV16 gene expression (Johansson et al., 2015).
2.4 Histone methylation
Unlike histone acetylation, involved exclusively in lysine gene activation, histone methylation can be involved in both gene activation and repression, which takes place on both lysine and arginine (Hsu et al., 2012). This process is generally performed by histone methyltransferases, specifically SET Domain Containing Lysine Methyltransferase 7 (SET7), Protein Arginine Methyltransferase 1 (PRMT1) and Coactivator-Associated Arginine Methyltransferase 1 (CARM1) (Hsu et al., 2012). Additionally, these histone methyltransferases are controlled by E6 oncoproteins, which determine whether the histones undergo methylation. In HPVs, a large variety of histone methylations can occur. For simplicity, only H3 and H4 histone methylations will be used as examples. For histone H3, a small amount of K4 is monomethylated, and the majority of K9, K27, and K36 are mono-, di- and tri-methylated (Porter et al., 2021). The methylation of H3K4 and H3K36 is typically associated with active chromatin, whereas the H3K9 and H3K27 methylation is associated with repressed chromatin. As for histone H4, K20 is primarily di-methylated. To summarize, a repressed chromatin results in reduced viral gene expression, whereas an active chromatin promotes the process.
2.5 DNA methylation
In HPVs, DNA methylation is mediated by histone methyltransferase. In the case of HPV16, the viral DNA can be regulated by methylation, which affects viral gene transcription (Zygouras et al., 2023). A key factor regulating the transcriptional activity as well as the replication of HPV16 genomes is the E2 protein, an important regulator of the viral E6 and E7 oncogene expression (Zygouras et al., 2023). The HPV16 genome contains four highly conserved E2-binding sites (E2BS) located near the DNA-binding sites. The binding of E2 to these various E2 binding sites activates or represses gene expression from the early promoter (Zygouras et al., 2023). The occupancy of the E2BS is partly controlled by the E2 levels. When E2 levels are high, the E2 protein binds to all of the E2BS. This binding then represses the activity of the early promoter and viral replication. Upon cellular differentiation, the three E2BS nearest the early promoter become methylated. This methylation prevents the E2 protein from binding and repressing the early promoter, allowing viral oncogenes E6/E7 to be expressed (Mac and Moody, 2020), thereby facilitating cervical carcinogenesis.
2.6 HPV16 E7 phosphorylation
E7 is one of the key oncoproteins contributing to the development of cervical cancer. It was found that the phosphorylation of Ser31 and Ser32 by casein kinase II (CKII) is crucial in regulating the activity of HPV16 E7, including its ability to transform cells and interact with TATA box-binding protein (TBP), which is a transcription factor (Nogueria et al., 2017). The role of CKII also allows E7 to have the ability to target the retinoblastoma tumor suppressor (pRb) and related p130 pocket proteins for degradation (Nogueria et al., 2017). As a result, increased levels of phosphorylation of a variant HPV-16 E7 also increases interaction with TBP and pRb, thereby facilitating HPV-induced malignancies.
3. Viruses: Influenza
Another virus that humans encounter frequently is Influenza, which has previously caused pandemics, such as those of 1918(H1N1 IAV pandemic), 1957 and 1968, and many more recent ones (Knobler et al ,2005).
3.1 Introduction to influenza
Influenza viruses have long been inextricably linked with the epigenome on many fronts, mainly in natural epigenomic variation in the human response, reaction to ‘epidrugs’ and in relation to the epigenetic change the virus itself can incite in the human. All of these describe how differences in the epigenome (specifically with DNA methylation and Histone tail modification) can elicit changes in the human immune response. Influenza is a virus of the family Orthomyxoviridae which is split into categories A, B, C and D, where Influenza A Virus (IAV), the most prominently investigated, is known mainly to cause pandemics. Influenza D is known to be found in animals, mainly cattle. Influenza B and C are both considered less severe, largely due to their inability to cause epidemics in general, although B causes seasonal outbreaks (CDC, 2021). IAV is classified using Hemagglutinin and Neuraminidase antigens, where the different combinations of the variations of the antigens are used to name different strains (i.e H1N1 strain – Hemagglutinin 1 and Neuraminidase 1)(Keshavarz et al., 2021). The principal foci of this section will be on H1N1 Swine Flu and Avian Flu H5N1- IAV variants which have caused pandemics, having been zoonotic.
3.2 Epigenetics and IAV
The influenza virus can interfere with several epigenetically regulated mechanisms in the cell, in an attempt to interfere with both general and specialized immunity as a part of the immune response (the specialized immune response is the adaptive immunity specific to the pathogen). An example of this would be the disturbance of the antigen presentation initiated by the H5N1 IAV strain (Avian Influenza virus). DNA methylation and histone tail modifications have been identified as the mechanism by which the process of antigen presentation (one which is vital in initiating adaptive immunity) is antagonised by H5N1 IAV (Menachery et al., 2018). There was evident down regulation of gene expression in genes pivotal to antigen presentation. A study by Menachery et al. focused on changes discovered in the Type-1 Interferon Stimulated genes (ISGs), which has its role in interfering in viral replication. It was found that ISGs were regulated by the H5N1 influenza, despite the cell’s functionality in terms of signaling and transcriptional activation factors (which are proteins aiding the activation of genes) being completely undamaged. It was the repressive histone markers associated with the down-regulated ISGs that confirmed the epigenetic change elicited by the virus itself. ISGs themselves are indirectly linked to antigen presentation and they provide the necessary genetic information to create Type 1 Interferons (IFNs), which have recently been shown to have a pivotal role in the regulation of MHC II restricted antigen, presenting pathways (those of antigen presentation in which the antigen of a foreign pathogen is displayed in the Major Histocompatibility Complex II on a cell surface) (Gessani et al., 2014). More directly, the IFN-y-associated antigen presentation gene’s expression was also down-regulated, providing comprehensive proof of the antagonistic actions of the H5N1 IAV in antigen presentation, through both DNA methylation and histone modification (Menachery et al., 2018). Proteomics (the study of proteomes which are groups of proteins) confirmed that there was consistent down regulation of antigen presentation genes, for example on the MHC locus on the human Chromosome 6 (Meenachery et al., 2018). This data displayed a statistically significant decrease (at 5% significance) in the creation of MHC proteins upon infection with the Avian Influenza (Menachery et al., 2018). There is still much to investigate on this topic, for example due to the same trend being non-existent when considering H1N1 Swine Flu strain of IAV (Menachery et al., 2018), but it seems fairly conclusive that the infection of an individual by IAV is likely to incite significant changes to the epigenome, thus leading to the immune system losing efficiency. Assuming a holistic view on this portion of epigenetic changes in relation to influenza, it becomes increasingly clear that evolution with respect to the virus itself, induced by selection pressures provided by adaptive human immunity, is what could have initiated the development of the virus in such a way that it can cause these epigenetic changes in its host.
The repression of the Type 1 IFN genes described above is caused by histone modification. The presence of the virus produced NS1 Protein (linked with viral transcription) which correlated with the increased presence of a repressive mark H3K27me3 (a trimethylated lysine which is the 27th amino acid on histone 3) as opposed to decreased H3K4me3, which is a mark for an active gene (Marcos-Villar et al., 2018). This silences the gene, eventually resulting in an antagonistic motion of reducing the production of IFN ( Liu et al., 2019). Furthermore, it prevents the JAK-STAT pathway from functioning properly; the JAK -STAT pathway is a vital cell-signaling system involved in reception of almost 40 cytokines (Liu et al., 2019). Although it hasn’t been completely ascertained how this works, it could be an epigenetic mechanism (Liu et al., 2019). It has been found that NS1 causes the methylase DNMT3B to dissociate from areas key in the maintenance and regulation of JAK-STAT signaling, proposing a possible mechanism (Liu et al., 2019) (Keshavarz et al., 2021). This is epigenetic: the methylase is responsible for varying levels of DNA methylation, which in turn varies gene expression.
3.3 Epidrugs
With a different perspective, some drugs can help use epigenetic changes in humans to fight the influenza virus (as well as other severe respiratory illnesses such as MERS-CoV or SARS-COV2). This is achievable through ‘epidrugs’, which are capable of reversing some epigenetic changes. Another option is also to interfere with some epigenetic pathways, which the viruses antagonize, with immune modulators able to supplement the immune system in its fight against a pathogen. An example of an epidrug is curcumin, a histone deacetylase inhibitor which is derived from turmeric (Crimi et al., 2020). Upon H1N1 infection, curcumin could decrease the secretion of proinflammatory cytokines which would induce severe, potentially unmanageable, symptoms of inflammation in the individual. It also downregulates the nuclear factor kappa-light-chain enhancer of activated B cell (NF-kB) gene in macrophages (Crimi et al., 2020). This is the set of proteins which controls expression of genes involved in inflammation (such as in cytokine production). These have epigenetic mechanisms (not directly changing the genes as they are externally introduced) and have positive impacts on the externalized symptoms induced by H1N1 IAV, and thus possess great potential in a care setting. Similarly, drugs which are commonly used in today’s world of medicine can also be repurposed as epidrugs due to their multi-beneficial properties. The best example of this would be statins, commonly used today to lower the production of LDL cholesterol in the body to mitigate or avoid diseases such as CVD. Statins have been found to have anti-inflammatory impacts and disrupt molecular pathways in the virus, minimizing tissue damage in the body and therefore proving that other alternatives are available to common treatment of the flu (Crimi et al, 2020). It was also found in a clinical trial with 31,989 participants (NCT01427309) (Diaz-Granados et al., 2014) that individuals who were both taking statins and had a high dose flu vaccine produced more antibodies than those with a normal dosage and no statins. Thus, epigenetic changes induced by drugs can affect the immune response of the body to the flu in a positive manner. Other epidrugs, such as Metformin, used commonly in the treatment of type 2 diabetes, also provide examples to this extent (Crimi et al., 2020).
4. Alzheimer’s
The development of Alzheimer’s Disease (AD) involves factors such as genetics, epigenetics, environment, lifestyle choices and ageing. AD is characterized by features such as senile plaques (made up of amyloid β (Aβ) neurofibrillary tangles within cells) and losses of synaptic connections and neurons. The E (APOE) ε4 allele has been linked to the development of AD in individuals with certain genetic risk factors (Madia Lozupone et al., 2023). Moreover, over thousands of years the human brain has evolved and adapted to perfectly modify DNA methylation and histone acetylation for optimal gene expression, thereby allowing humans to thrive in a variety of environments. If we are able to trace the evolutionary roots of this disease, there may lie vital insights and knowledge into the complex pathogenesis of Alzheimer’s.
4.1 Epigenetic Mechanisms present in Alzheimer’s
One of the key epigenetic mechanisms in patients suffering from AD is DNA methylation – the process of adding methyl groups to DNA. Aberrant DNA methylation patterns have been observed in Alzheimer’s patients, most commonly found in genes involved in neural function (De Plano et al., 2024).
An example of this decreased methylation is found in a gene that codes for the amyloid precursor protein (APP) (Liu, Jiao and Shen, 2018). This in turn will result in an increase in the expression of APP which plays an important role in forming the amyloid-beta plaques found in the brains of people affected by Alzheimer’s (Yokoyama, Rutledge and Medici, 2017).
4.2 Lifestyle and Environmental Risk Factors that contribute to AD
Certain lifestyle and environmental factors that play a role in epigenetic mechanisms have also been linked as AD risk factors. Air pollution, diet, level of activity, and mental health issues are examples of important factors to consider due to their role in inducing epigenetic changes that may contribute to Alzheimer’s development and progression (Nicolia, 2015).
An exposure to environmental toxins or air pollutants has been linked to certain epigenetic mechanisms, such as the alteration of DNA methylation patterns in the brain that can increase the risk of developing AD. This study found that in mice there was a clear link between “APOE network, air pollution, and age-related diseases” (Haghani et al., 2020). Furthermore, “human and mouse shared 87% of potential binding sites for transcription factors in APOE cluster promoter, suggesting similar inducibility and a novel link between environment, APOE cluster and risk of AD.”(Haghani et al., 2020).
Another factor that could influence the epigenetic modifications linked to AD is one’s diet (Sezgin and Dincer, 2014). Specific nutrients like folate, vitamin B-12 and omega-3 fatty acids are vital for maintaining proper methylation levels of your DNA (Allison et al., 2021). A deficiency in these nutrients and minerals can result in a disruption in the epigenetic regulation of certain genes. In this study it was seen that adopting a mediterranean diet for one year can lead to epigenetic rejuvenation in elderly subjects, however there were “country-, sex-, and individual-specific effects”(Gensous et al., 2020), thus highlighting that a therapeutic approach will require personalized methods of action.
4.3 Therapeutic Interventions addressing epigenetic modifications in Alzheimer’s disease
The significance of environmental exposures to the epigenome is well evidenced. Given the importance of the role of epigenetic modifications on the development of AD, drugs and therapies that target these mechanisms propose a promising strategy for preventative measures.
One example of a possible therapeutic intervention that could help prevent AD are compounds inhibiting histone deacetylase (HDAC) such as valproic acid or sodium butyrate (Vecsey et al., 2007). These compounds have been able to restore normal gene expression in mice, thus through modification of these epigenetic regulators there is real promise for a true prevention of AD in the future. On the other hand, the interventions that target DNA methylation could also be a possible solution for the prevention of AD. In a recent preclinical trial (Yokoyama, Rutledge and Medici, 2017), DNMT inhibitors have been shown to possess the ability to reduce neurodegeneration related to AD. In summary, epigenetic changes play a very significant role in the development of AD, alongside environmental and lifestyle factors like air pollution, exposure to pollutants, diet and mental health issues. Targeting these mechanisms promotes a promising approach for Alzheimer’s prevention and treatment.
5. Lung cancer
Cancer has been treated as a genetic disease, primarily focusing on genetic mutations and the impacts of external factors. Studies have shown that cancer cells often display epigenetic modifications, suggesting that these changes play a crucial role in cancer development. Epigenetic association has been made with lung cancer, as changes in DNA methylation and histone modifications can alter gene expression, resulting in the development of oncogenes.
5.1 Gene-specific (DNA methylation)
DNA methylation in particular plays a critical role in regulating gene expression and maintaining genomic stability. In cancer cells, mutations often occur in cytosine-guanine dinucleotides (CpG sites), which are responsible for genome stability. This is a genetic change, which may result in epigenetic change depending on the impacted gene. Hypomethylation, where there is a decreased amount of methylation, of CpG islands results in the suppression of certain genes that are meant to be active, and conversely, certain genes that are meant to be suppressed become active. In cancer, genes which become more active include HER2 gene, which helps cancer grow (Gutierrez et al., 2011). An example of a gene that becomes suppressed is BRAF kinase, an enzyme which helps with cell expression (El-Telbany et al., 2012). Moreover, this is seen in lung cancer as genetic mutations in CPG sites which code for DNA methyltransferases (enzymes which add methyl groups) can lead to the progression of cancer due to the altered methylation patterns. This dysregulation can lead to the activation of oncogenes, which promotes abnormal cell growth and can contribute to cancer development (Ansari et al., 2016). This dysregulation of transcriptional processes, such as apoptosis, metastasis and epithelial-mesenchymal transition (EMT), is a hallmark of cancer progression (Ansari et al., 2016). Considering this, DNA methylation is an exceptional epigenetic marker. Thus, alterations in DNA can lead to cancer in a variety of ways. For example, hypermethylation of the promoter region on the p16INK4a gene, which is rich in CpG, leads to a silencing of the gene recorded to contribute to lung cancer progression (Liyanaged et al., 2019). Smoking is associated with the production of carcinogens that can cause alterations in the expression of DNA methyltransferase 1, hence a lack of methyl groups are added, thereby activating genes like HER2. Carcinogens are substances capable of causing cancers, for example radon gas and asbestos. The expression of DNA methyltransferase 1 (DNMT1) increases in smokers due to inducing factors such as tobacco, and processes like post-translational modification (Ansari et al., 2016). It has been proposed that epigenetic modifications may be inheritable via transgenerational inheritance. Transgenerational inheritance is the transfer of epigenetic markers from one generation to the next. Epigenetic markers prominent in lung cancer, such as the BRCA1 gene, may be inherited by offspring, leading to an increased susceptibility of developing similar or related conditions (SB et al., 2019). Thus, understanding the role of DNA methylation and other epigenetic changes is crucial for understanding potential cancer causes.
5.2 Histone modification
Histone modifications can occur for a number of reasons including genetic mutations, environmental influences, and stress responses. The epigenome of many cancer cells commonly possess enzymes called histone deacetylation (HDAC) (Audia et al., 2016). The increased activity of HDACs has marked a loss of several histone markers, altering the cells. Many epigenetic therapies have found similar histone modifications in the genome from parent to offspring. For example, histone methyltransferases (HMTs), which add methyl groups to histones, are commonly altered, placing the methyl group in the wrong areas and causing the activation or repression of certain markers (Bajbouj et al., 2021). This is also seen with histone demethylases (HDMs), responsible for removing methyl groups. In lung cancer, histone modification is prominent through the methylation histone H3 at lysine 27 (H3K27me3) by the enzyme EZH2, therefore leading to gene silencing (Sharma S et al., 2010). When certain genes meant to be expressed or suppressed are altered due to histone modifications, essentially an abnormal chromatin structure is developed resulting in oncogene development.
5.3 Introduction to lung cancer and epigenetics
As seen with certain genes such as p16INK4a, markers have assisted in proving epigenetic traits in cancer. These markers have commonly been present in the parent and have been passed down to their offspring with limited presence in their epigenome. The intergenerational inheritance has shown that many cancers are highly heritable from one generation to the next (Goldgar et al., 1994). For example, the KDM6A protein has histone demethylase activity toward lysine 27 on histone H3 (H3K27). KDM6A is a histone demethylase enzyme that targets the lysine 27 residue on histone H3 (H3K27) and it plays a crucial role in gene expression regulation. In a study conducted by Hong et al., the gene critical for the demethylation of lysine 27 (Kdm6a) was deleted,. If lysine 27 is not suppressed it could cause lung cancer, hence why the offspring of the male had tumors present in the next two generations (Hong et al.. 2019). This study helps confirm that epigenetic markers can influence the progression of cancer, specifically lung cancer.
Moreover, the familial link was established from another study in which non-small cell lung cancer (NSCLC) was found in individuals who were both smokers and non-smokers. However, 18% of 230 NSCLC patients who had never smoked had familial lung cancer (Gaughan EM et al., 2017). In another study, there was more than a five-fold higher chance of an individual whose family was exposed to lung cancer to have two copies of the high-risk alleles rs8034191 and rs1051730, both markers of lung cancer (Liu et al., 2018).
In summary, through exploring epigenetics’ involvement in lung cancer treatments, further research can be conducted. Understanding the mechanisms behind epigenetics can assist with preventative measures. The future of lung cancer can include epigenetic therapies, such as epidrugs, to further minimize susceptibility to oncogenes. For example, DNMT inhibitors, such as azacytidine, can be used to activate repressed genes in order to prevent oncogene development. Similarly, methylation marks can be used for early detection and prognosis (Anasari et al., 2016).
6. Epigenetics from an Evolutionary Perspective
This section presents the evolutionary origins and evaluates the evolutionary implications of epigenetics. Although it would be better if this section focused on the human species, due to a limited research database on humans, references are made mainly to studies on other model animals. Caution must be made when drawing conclusions for species that are not so closely related. We have judged that a reference to the implications of the existence of transgenerational epigenetic inheritance is mandatory, although we recognize that research is still inconclusive as to whether it exists in our species. This article’s purpose is not to evaluate that.
6.1 The origin of epigenetic mechanisms
Recently, epigenetic studies showed the conservation of molecules involved in mediating epigenetic modification, such as methyltransferases, across deep diverse branching species, including stramenopiles, plants, and animals, suggesting a fundamental role in shaping species genomes across different scales of evolutionary time.
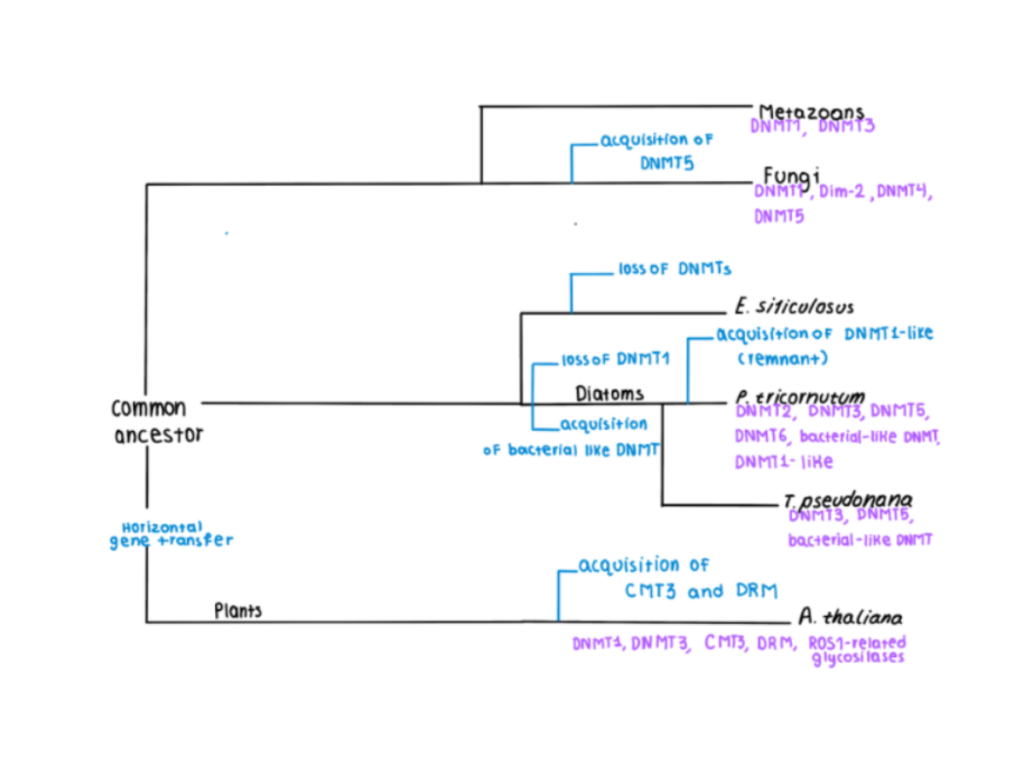
Figure 4: A phylogenetic tree showing the evolutionary pathway of the acquisition of DNA methyltransferases (DNMTs) for specific species. Data for the making of this figure was collected from Rastogi A. et al., 2015.
DNA methylation mechanisms are in general common to all three kingdoms. The methylation of cytosine, more specifically, appears to be the most conserved amongst the animal kingdom, due to its involvement in X-chromosome inactivation, transposable element silencing, gene expression regulation, and imprinting mechanisms. The only recorded organisms that show little to no DNA methylation employment are some yeast species, Drosophila melanogaster, Caenorhabditis elegans, and Ectocarpus siliculosus, but this appears to be a trait that evolved separately from these species.
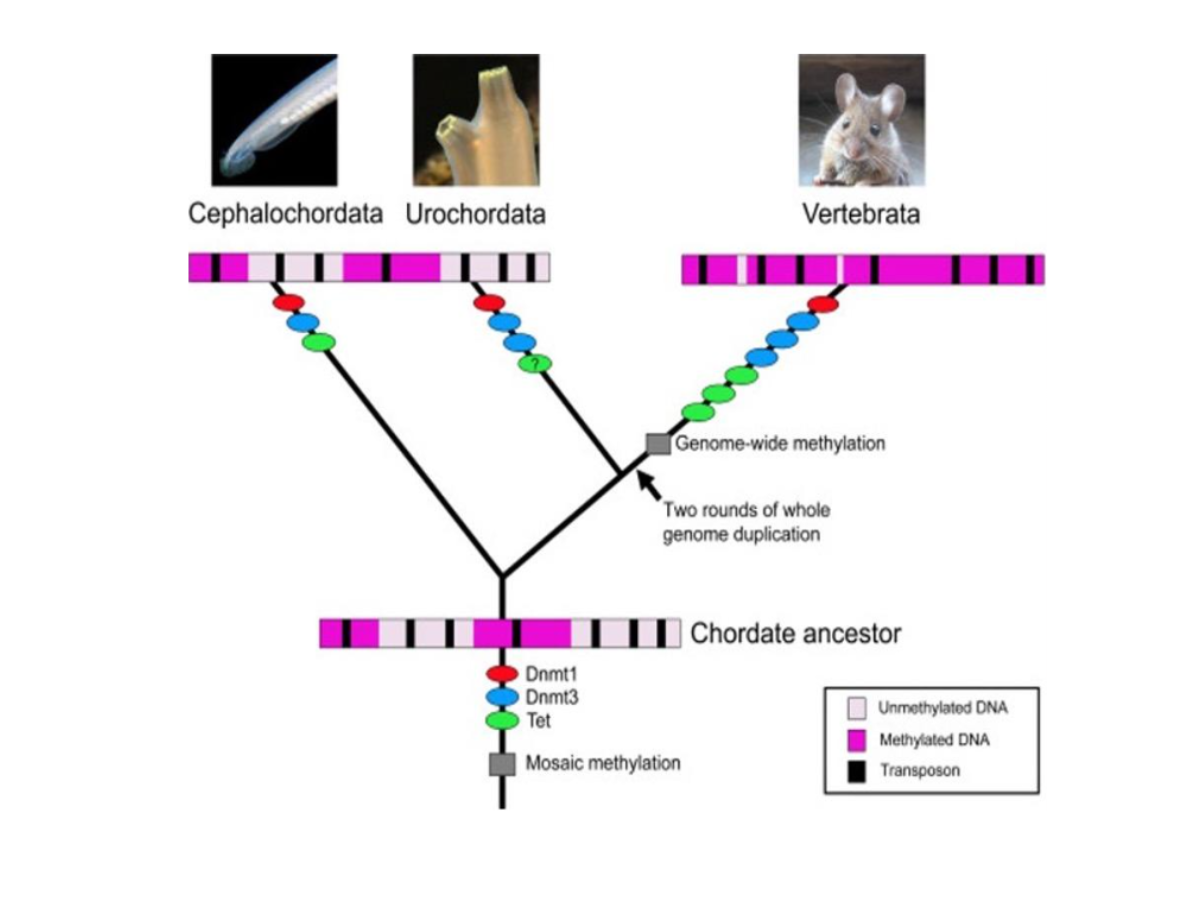
Figure 5: A phylogenetic tree showing the acquisition of two types of DNMTs and ten-eleven translocation proteins involved in DNA demethylation. Figure taken from Günter Vogt, 2023, Evolution, Functions and Dynamics of Epigenetic Mechanisms in Animals, Chapter 27.
Histones and histone modification are not as widespread, seeing as the bacteria domain has no histones. The structure of the nucleosome however, is similar amongst Eukaryotes and Archaea. This similarity and the correlation, investigated by Ammar R. et al. between nucleosome occupancy and gene expression in archaea suggest that nucleosomes and chromatin initially functioned to regulate gene expression, rather than just package DNA, highlighting the evolutionary importance of histone modification. Further mass spectrometry analysis of histones in Phaeodactylum tricornutum, mammals, and plants, which possessed epigenetic markers, indicated the early divergence of important Post Translational Modifications (PTM) (Veluchamy A. et al., 2015).
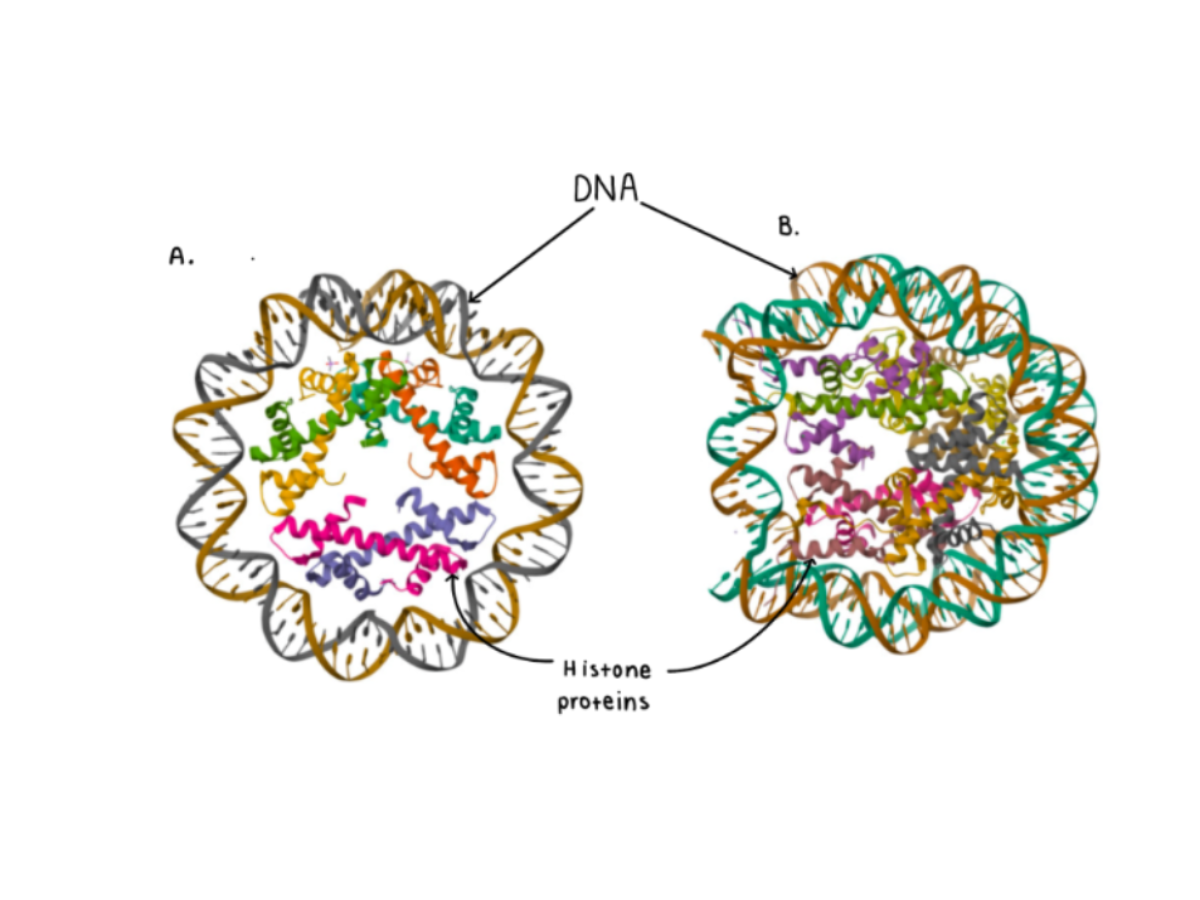
Figure 6: A crystal structure comparison of the Archael nucleosome (A) and the Human nucleosome (B). Structure resource: Protein Data Bank (PDB), A: PDB file entry 5T5K, https://www.rcsb.org/structure/5t5k; B: PDB file entry 2CV5, https://www.rcsb.org/structure/2cv5.
Non-coding RNAs are present in all kingdoms. Most of this previously called “Junk DNA” has no known function, but it appears that a great part of it is involved in gene expression, including epigenetic regulation mechanisms. It appears that ncRNAs are involved mainly in gene silencing and can act as templates for the reconstruction of primary epigenetic signals, thus acting as secondary signals in epigenetic regulation (Fitz-James M.H. and Cavalli G., 2022). Throughout the evolutionary passage, the percentage of ncRNA has changed differently for different organisms, with more complex ones obtaining a greater percentage (Rastogi A. et al., 2015).
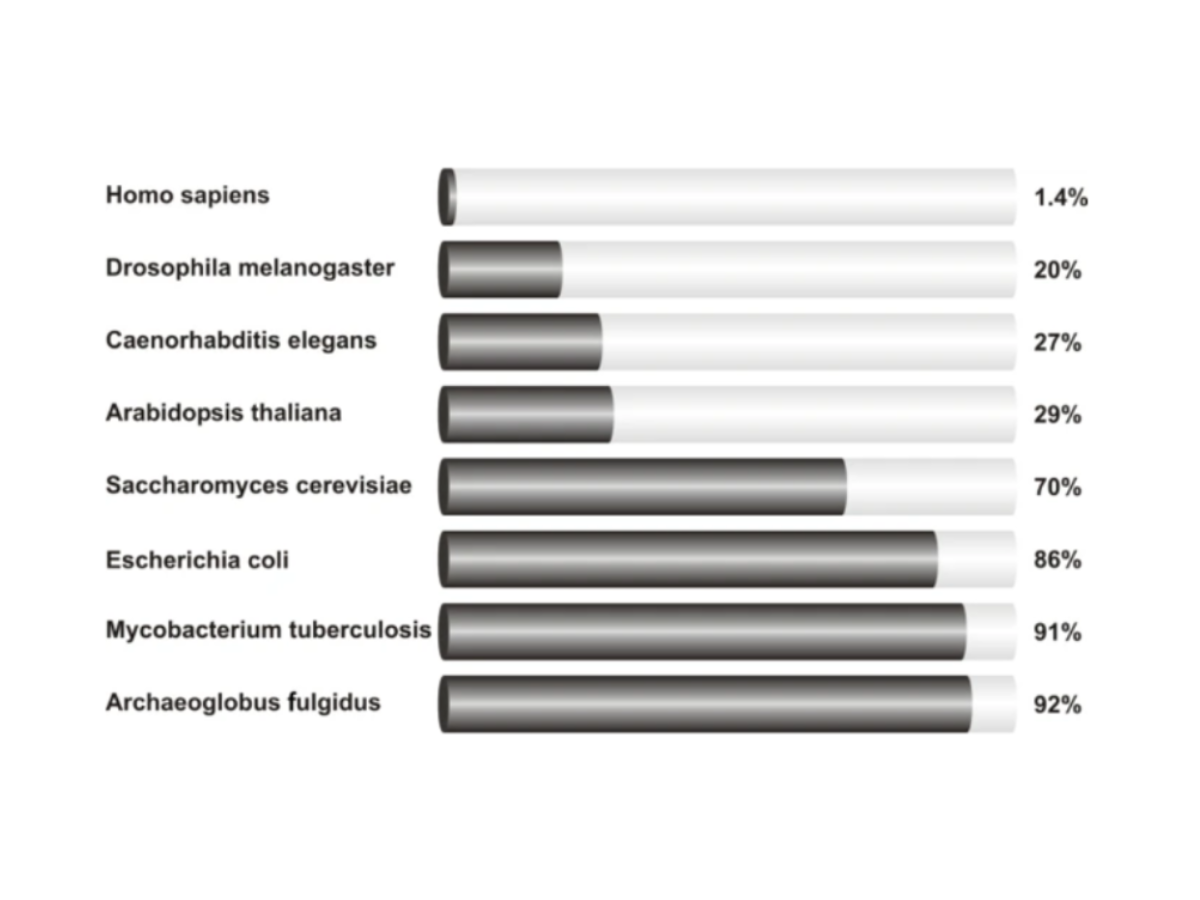
Figure 7: The percentage of protein-coding genes sequences in several eukaryotic and bacterial genomes. Image and description taken from Sana J. et al., 2012.
Comparative genomics and molecular biology studies often reveal that many ncRNAs have deep evolutionary roots, further supporting their presence in LUCA (Igor Ulitsky, 2016). Whether the epigenetic modificatory-type ncRNAs are the ones persisting for so long in the evolutionary race is still inconclusive. Igor Ulitsky and David P. Bartel have argued that some of the remnant ncRNA has no fitness advantage, and is maintained by the cell mechanisms as it is a better option rather than trying to prevent their production through more stringent control mechanisms with potential to evolve. It is unclear whether this applies to ncRNAs active in epigenetic modification mechanisms, however, it is a supported evolutionary origin of some epigenetic mechanisms.
6.2 I. Adaptation and Survival
In their article, Zhang Y.-Y. et al. (2018) suggest that “the study of evolution and adaptation may be incomplete without taking heritable epigenetic variation into account”.
It is thought that epigenetic inheritance (transgenerational or not) can facilitate the process of adaptation in two main ways: firstly by offering immediate adaptive benefits, more rapidly than if the population was relying on genetic variation alone (Kronholm I. and Collins S., 2016; Burggren W., 2016) and secondly due to the rate of creation of epigenetic variation being higher than the mutation rate (Der Graaf A. et al., 2015). These two “properties” of epigenetic inheritance are especially important where limited genetic diversity in a population is involved – a case when the population puts up against a serious survival pressure. This is true especially when it comes to invasive species. On this topic, Christina Richards, an evolutionary ecologist at the University of South Florida in Tampa, says: “There are a lot of different ways for invasive species to do well in novel environments and I think epigenetics is one of those ways” (Gupta S., 2013). Research on the invasive species of Passer domesticus (by Liebl A., findings summarized in Liebl A et al., 2015) illustrates this view. The group of birds studied had very little genetic diversity. But, when it came to their methylome, a high variability was found. These findings are notable due to the relationship between the population’s epigenetic variation and high rate of adaptability to new environments, which may imply the existence of a link between those two.
What is also interesting is the link between epigenetics and inbreeding depression, which acts as a mechanism to prevent the survival of individuals with high homozygosity index, thus aiding in increasing the gene pool of the population. Because the majority of studies show higher incidences of methylation in inbred individuals and often resulting in gene silencing, this situation is likely connected to lower fitness in inbred individuals. For example, a study of the plant Scabiosa columbaria found that chemical demethylation alleviated inbreeding depression, i.e. gene silencing due to epigenetic modifications, was causing the difference in fitness between inbred and outbred individuals (Vergeer et al., 2012). This suggests that inbreeding depression can be of environmental impact and not exclusively in genetic mutations, in comparison with the traditional view of inbreeding, and that a large part of the evolutionary purpose of epigenetics remains still not fully understood.
To evaluate the evolutionary significance of epigenetic variation, researchers (Zhang Y.-Y. et al., 2018) compared heritable phenotypic variation in epigenetic recombinant inbred lines (epiRILs) of Arabidopsis thaliana, that mainly differ in DNA methylation, with genetic RILs and natural ecotypes in order to assess growth, phenology, and fitness under common conditions. The data showed that heritable phenotypic variation in epiRILs of A. thaliana has been measured at a level comparable with that observed in genetic RILs and natural ecotypes under the same conditions. DNA methylation differences corresponded to variation among epiRILs. Life-history patterns of the Met1-epiRILs separated into two distinct types, reminiscent of the wild populations, suggesting an evolutionary role for epigenetic variation. The authors emphasized that epigenetic variation could provide a source of stable phenotypic diversity of considerable extent, but further molecular analysis is required to validate these findings.
6.2 II. Speciation
The adaptable quality of epigenetics, as previously explained, can result in reproductive isolation, a key factor in speciation. For instance, differential methylation patterns between populations can lead to incompatibilities in hybrid offspring, reducing their viability or fertility and promoting speciation (Bonduriansky and Day, 2009). Furthermore, epigenetic modifications can stabilize hybrid genomes in newly formed species, facilitating the establishment of unique traits and enhancing species divergence (Madlung and Comai, 2004). In addition, a comparative analysis of the DNA methylation status between humans and great apes was carried out by Hernando-Herraez I. et al. (2013). CpG methylation profiles were generated using Illumina Methylation450 bead arrays in nine human samples and 23 great ape samples. One of the most surprising findings was that the phylogenetic tree of the great apes studied could be generated using methylation data alone.
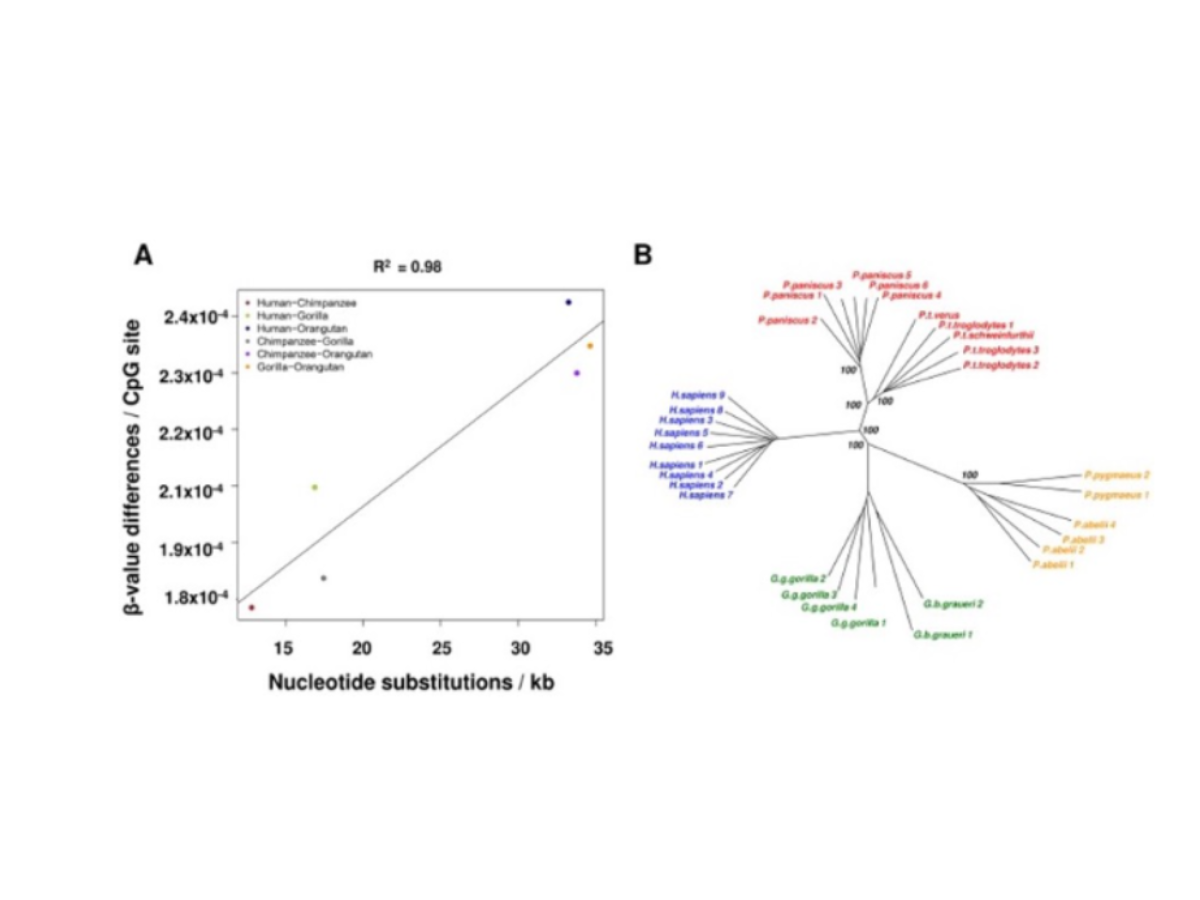
Figure 8: Some of the results of the investigation carried out by Hernando-Herraez et al., 2013. (A) Methylation changes correlate with DNA sequence changes: x-axis shows the number of nucleotide substitutions between two species per kb; y-axis shows the changes in methylation based on β-values. (B) Neighbor-joining tree based on methylation data from probes with a perfect match in all references genomes (31,853 autosomal CpGs). Bootstrap values (1,000 permutations) are shown for each node. The diagrams and the descriptions belong to the authors, Hernando-Herraez et al.
Even though all these data provide solid proof for the possible link between adaptability and epigenetics, it is essential to recognize that this adaptive value is not the same in all cases. It is logical to think that organisms that would benefit the most from this are those who live in a slowly fluctuating environment. This means for our purpose that the length of generation time is smaller than the cycle length of environmental conditions, so the conditions experienced by the parents are a reliable indicator of the conditions the offspring will live (Burgess and Marshall, 2014; Jablonka and Raz, 2009; Uller et al., 2015). Therefore, it can be concluded that organisms with short life cycles would benefit more than organisms with larger ones (Houri- Zeevi and Rechavi, 2017).
We must also be open to objections to the evolutionary advantage of epigenetic inheritance, since it has been suggested that it is of high metabolic demand to care for the production and maintenance of an effective epigenetic machinery (Macartney et al., 2018).
To sum up, epigenetic mechanisms create genetic variation and aid in rapid evolution and survival through gene expression changes due to environmental circumstances. They also affect the speciation process through reproductive isolation and hybrid stabilization. Some studies suggest that epigenetic variability explains human evolution and adaptation of invasive species. This generally makes epigenetics a dynamic tool for species’ adaptation, survival, and evolution, highlighting a close interrelation between genetics and the environment, and explains why even though similar mechanisms hold the keys to the progression of autoimmune diseases, such as Alzheimer’s and tumor progression, as previously demonstrated, epigenetic regulation has not been removed from the majority of population gene pools.
7. Conclusion
To summarize, epigenetics is a crucial factor in health and disease, working through mechanisms such as histone modification, histone acetylation, and DNA methylation. These processes regulate gene expression without altering the DNA sequence itself. Understanding how epigenetics influences health can lead to more effective, personalized treatments that target the underlying causes of diseases.
Viruses can manipulate the host’s epigenetic machinery to evade the immune system and persist in the body. Viruses like human papilloma virus and influenza have showcased epigenetic properties through the monitored DNA sequences showcasing chromatin remodeling, and histone modifications. Studying these interactions is essential for creating better treatments.
Epigenetics also plays an important role in Alzheimer’s disease: epigenetic modifications can affect the expression of genes involved in neurodegeneration and memory loss. Abnormal DNA methylation and histone modifications have been linked to the progression of Alzheimer’s, allowing the development of treatments to reverse cognitive decline.
In cancers like lung cancer, epigenetic changes can disrupt normal gene regulation, leading to uncontrolled cell growth and the formation of tumors. Transgenerational inheritance of epigenetic traits showcases the heritability of cancer susceptibility, with specific gene modifications being passed down from parent to offspring. Studies demonstrating the hypermethylation of the p16INK4a promoter region and the impact of histone modifications like H3K27me3 in lung cancer exemplify how epigenetic dysregulation contributes to oncogenesis. These epigenetic changes, such as the hypermethylation of CpG islands leading to the silencing of tumor suppressor genes or the activation of oncogenes through hypomethylation, play a crucial role in the aberrant cellular behavior characteristic of cancer. Smoking-induced epigenetic changes further highlight environmental influences on gene expression and cancer risk. By identifying these specific changes, researchers can develop new strategies for early detection and targeted therapies aimed at addressing the root causes of cancer. Epigenetics also plays an important role in evolution. It allows organisms to adapt to environmental changes through non-genetic modifications, providing a way for species to adjust and survive in changing conditions. This insight helps us understand how evolution works and how organisms respond to their environments over time.
Overall, ongoing research in epigenetics holds great promise for developing new preventative measures and treatments. By uncovering the complexities of these processes, we can improve health outcomes, develop targeted therapies, and gain a deeper understanding of how life adapts and evolves.
Bibliography
Allison, J., Kaliszewska, A., Uceda, S., Reiriz, M. and Arias, N. (2021). Targeting DNA Methylation in the Adult Brain through Diet. Nutrients, 13(11), p.3979. doi: https://doi.org/10.3390/nu13113979
Ammar R., Torti D., Tsui K., Gebbia M., Durbic T., Bader G. D, Giaever G., Nislow C. (2012): Chromatin is an ancient innovation conserved between Archaea and Eukarya. Available online at: https://elifesciences.org/articles/00078
Anderson, O.S., Sant, K.E. and Dolinoy, D.C. (2012). Nutrition and epigenetics: an interplay of dietary methyl donors, one-carbon metabolism and DNA methylation. The Journal of Nutritional Biochemistry, [online] 23(8), pp.853–859. doi: https://doi.org/10.1016/j.jnutbio.2012.03.003
Ansari, J., Shackelford, R.E. and El-Osta, H. (2016). Epigenetics in non-small cell lung cancer: from basics to therapeutics. Translational Lung Cancer Research, 5(2), pp.155–171. doi: https://doi.org/10.21037/tlcr.2016.02.02
Aracena, K.A., Lin, Y.-L., Luo, K., Pacis, A., Gona, S., Mu, Z., Yotova, V., Sindeaux, R., Pramatarova, A., Simon, M.-M., Chen, X., Groza, C., Lougheed, D., Gregoire, R., Brownlee, D., Boye, C., Pique-Regi, R., Li, Y., He, X. and Bujold, D. (2024). Epigenetic variation impacts individual differences in the transcriptional response to influenza infection. Nature Genetics, 56(3), pp.408–419. Available online at: https://doi.org/10.1038/s41588-024-01668-z
Audia JE, Campbell RM. Histone Modifications and Cancer. Cold Spring Harb Perspect Biol. 2016 Apr 1;8(4):a019521. doi: 10.1101/cshperspect.a019521. PMID: 27037415; PMCID: PMC4817802.
Bajbouj K, Al-Ali A, Ramakrishnan RK, Saber-Ayad M, Hamid Q. Histone Modification in NSCLC: Molecular Mechanisms and Therapeutic Targets. Int J Mol Sci. 2021 Oct 28;22(21):11701. doi: 10.3390/ijms222111701. PMID: 34769131; PMCID: PMC8584007.
Banta J.A., Richards C.L. (2018): Quantitative epigenetics and evolution. Heredity 121, 210–224. Available online at: https://doi.org/10.1038/s41437-018-0114-x
Baylin SB, Jones PA. Epigenetic Determinants of Cancer. Cold Spring Harb Perspect Biol. 2016 Sep 1;8(9):a019505. doi: 10.1101/cshperspect.a019505. PMID: 27194046; PMCID: PMC5008069.
Bekdash, R.A. (2023). Methyl Donors, Epigenetic Alterations, and Brain Health: Understanding the Connection. International Journal of Molecular Sciences, 24(3), pp.2346–2346. doi: https://doi.org/10.3390/ijms24032346
Bhattacharyya, S., Mattiroli, F., Dyer, P.N., Sandman, K., Reeve, J.N., Luger, K. Protein Data Bank (PDB), 2017: Structure of histone-based chromatin in Archaea, file entry name: 5T5K, Available online at: https://www.rcsb.org/structure/5t5k
Bird A.,(2007): Perceptions of epigenetics. Nature, 447(7143), 396-398. Available online at: https://www.nature.com/articles/nature05913
Bonduriansky R. & Day T. (2009): Nongenetic Inheritance and Its Evolutionary Implications, Annual Review of Ecology, Evolution ,and Systematics Vol. 40: pp 103-125, Available online at: https://www.annualreviews.org/content/journals/10.1146/annurev.ecolsys.39.110707.173441
Bonduriansky R., Crean A. J and Day T. (2011): Evolutionary Applications: The implications of nongenetic inheritance for evolution in changing environments. Available online at: https://www.ncbi.nlm.nih.gov/pmc/articles/PMC3353344/
Borges B., (2022): Epigenetic alterations in canine mammary cancer, Genetics and Molecular Biology 45(3 suppl 1)45(3 suppl 1), Available online at: https://www.researchgate.net/publication/364705421_Epigenetic_alterations_in_canine_mammary_cancer
Burgess S. C. and Marshall D. J., (2014): Adaptive parental effects: the importance of estimating environmental predictability and offspring fitness appropriately, Oikos Volume 123, Issue 7 p. 769-776. Available online at: https://nsojournals.onlinelibrary.wiley.com/doi/10.1111/oik.01235
Burggren W. (2016): Epigenetic inheritance and its role in evolutionary biology: re-evaluation and new perspectives. Biology (Basel), 5(2), p.24. Available online at: https://doi.org/10.3390/biology5020024
Burley, M., Roberts, S. and Parish, J.L (2020). Epigenetic regulation of human papillomavirus transcription in the productive virus life cycle. Semin Immunopathol, 42, pp.159–171 (2020). Available at: https://doi.org/10.1007/s00281-019-00773-0
Cacabelos, R. and Torrellas, C. (2015). Epigenetics of Aging and Alzheimer’s Disease: Implications for Pharmacogenomics and Drug Response. International Journal of Molecular Sciences, [online] 16(12), pp.30483–30543. doi: https://doi.org/10.3390/ijms161226236
Centers for Disease Control and Prevention (2021). Types of Influenza Viruses.Centers for Disease Control and Prevention. Available at: https://www.cdc.gov/flu/about/viruses/types.htm.
Chao YL, Pecot CV. Targeting Epigenetics in Lung Cancer. Cold Spring Harb Perspect Med. 2021 Jun 1;11(6):a038000. doi: 10.1101/cshperspect.a038000. PMID: 32900703; PMCID: PMC8168531.
Chen, X., Yu, S. and Fan, J. (2018). Epigenetic diagnostic biomarkers for non-small cell lung cancer: present and future perspectives. Translational Cancer Research, 7(3), pp.796–802. doi: https://doi.org/10.21037/tcr.2018.05.03
Cheptou P-O., Donohue K. (2013): Epigenetics as a new avenue for the role of inbreeding depression in evolutionary ecology. Heredity 110, 205–206. Available online at: https://doi.org/10.1038/hdy.2012.66
Crimi E, Benincasa G, Figueroa-Marrero N, Galdiero M ,Napoli C (2020)Epigenetic susceptibility to severe respiratory viral infections and its therapeutic implications: a narrative review. British Journal of Anaesthesia, 125(6), pp1002-1017. Available online at: https://www.bjanaesthesia.org/article/S0007-0912(20)30563-8/fulltext
De Lorenzi R., (2020): New Insights into epigenetic modifications, EMBL. Available online at: https://www.embl.org/news/science/new-insights-into-epigenetic-modifications/
De Plano, L.M., Saitta, A., Oddo, S. and Caccamo, A. (2024). Epigenetic Changes in Alzheimer’s Disease: DNA Methylation and Histone Modification. Cells, [online] 13(8), p.719. doi: https://doi.org/10.3390/cells13080719.
Der Graaf A., Wardenaar R., Neumann D. A., Taudt A., Shaw R. G., Jansen R. C., Schmitz R. J., Maria Colomé-Tatché, and Johannes F. (2015): Rate, spectrum, and evolutionary dynamics of spontaneous epimutations. Proceedings of the National Academy of Sciences of the United States of America, 112(20), pp.E3006-E3015. Available online at: https://www.pnas.org/doi/full/10.1073/pnas.1424254112
DiazGranados, C.A., Dunning, A.J., Kimmel, M., Kirby, D., Treanor, J., Collins, A., Pollak, R., Christoff, J., Earl, J., Landolfi, V., Martin, E., Gurunathan, S., Nathan, R., Greenberg, D.P., Tornieporth, N.G., Decker, M.D. and Talbot, H.K. (2014). Efficacy of High-Dose versus Standard-Dose Influenza Vaccine in Older Adults. New England Journal of Medicine, 371(7), pp.635–645. Available online at: https://doi.org/10.1056/nejmoa1315727
Dong, W, Wang, H, Li, M, Li, P and Ji, S (2024) Virus-induced host genomic remodeling dysregulates gene expression, triggering tumorigenesis. Available at: https://doi.org/10.3389/fcimb.2024.1359766
Du, C., Tan, L., Xiao, X., Xin, B., Xiong, H., Zhang, Y., Ke, Z. and Yin, J. (2024). Detection of the DNA methylation of seven genes contribute to the early diagnosis of lung cancer. Journal of Cancer Research and Clinical Oncology, 150(2). doi: https://doi.org/10.1007/s00432-023-05588-z
El-Telbany A, Ma PC. Cancer genes in lung cancer: racial disparities: are there any? Genes Cancer. 2012 Jul;3(7-8):467-80. doi: 10.1177/1947601912465177. PMID: 23264847; PMCID: PMC3527990.
Feil R. and Fraga M. F. (2012): Epigenetics and the environment: emerging patterns and implications,Nature Reviews Genetics, Available online at: https://www.nature.com/articles/nrg3142
Ferguson, J, Leona, K.C, Pentlanda, I, Stocktonb, J, Güntherc, T, Beggsab, A, Robertsa, S, Noyvertad, B and Parish, J.L (2021). The chromatin insulator CTCF regulates HPV18 transcript splicing and differentiation-dependent late gene expression. Available at: https://doi.org/10.1101/2021.04.30.442078
Feschotte C. (2008): Transposable elements and the evolution of regulatory networks. Nature Reviews Genetics, 9(5), 397-405. Available online at: https://www.ncbi.nlm.nih.gov/pmc/articles/PMC2596197/
Fitz-James M.H., Cavalli G. (2022): Molecular mechanisms of transgenerational epigenetic inheritance. Nat Rev Genet 23, 325–341 Available online at: https://doi.org/10.1038/s41576-021-00438-5
Gensous, N., Garagnani, P., Santoro, A., Giuliani, C., Ostan, R., Fabbri, C., Milazzo, M., Gentilini, D., di Blasio, A.M., Pietruszka, B., Madej, D., Bialecka-Debek, A., Brzozowska, A., Franceschi, C. and Bacalini, M.G. (2020). One-year Mediterranean diet promotes epigenetic rejuvenation with country- and sex-specific effects: a pilot study from the NU-AGE project. GeroScience, 42(2), pp.687–701. doi: https://doi.org/10.1007/s11357-019-00149-0
Gessani, S., Conti, L., Del Cornò, M. and Belardelli, F. (2014). Type I Interferons as Regulators of Human Antigen Presenting Cell Functions. Toxins, 6(6), pp.1696–1723. Available at: https://doi.org/10.3390/toxins6061696.
Günter Vogt, Chapter 27 – Evolution of Epigenetic Mechanisms in Animals and Their Role in Speciation, Handbook of Epigenetics (Second Edition); (2017), Academic Press, Pages 409-426, ISBN 9780128053881, Available online at: https://doi.org/10.1016/B978-0-12-805388-1.00027-4.
Gupta S. (2013): Epigenetics posited as important for evolutionary success. Nature. Available online at: https://doi.org/10.1038/nature.2013.12179
Haghani, A., Thorwald, M., Morgan, T.E. and Finch, C.E. (2020). The APOE gene cluster responds to air pollution factors in mice with coordinated expression of genes that differs by age in humans. Alzheimer’s & Dementia, [online] 17(2), pp.175–190. doi: https://doi.org/10.1002/alz.12230
Hernando-Herraez I. , Prado-Martinez J. , Garg P., Fernandez-Callejo M., Heyn H., Hvilsom C., Navarro A., Esteller M., Sharp A. J. and Marques-Bonet T. (2013): Dynamics of DNA Methylation in Recent Human and Great Ape Evolution, PLOS Genetics, 9(9), e1003763. Available online at: https://doi.org/10.1371/journal.pgen.1003763
Hoang, P.H. and Landi, M.T. (2022). DNA Methylation in Lung Cancer: Mechanisms and Associations with Histological Subtypes, Molecular Alterations, and Major Epidemiological Factors. Cancers, 14(4), p.961. doi: https://doi.org/10.3390/cancers14040961
Houri-Zeevi L., and Rechavi O. (2017): A Matter of Time: Small RNAs Regulate the Duration of Epigenetic Inheritance. Trends in genetics : TIG, 33(1), 46–57.Available online at: https://doi.org/10.1016/j.tig.2016.11.001
Hsu, C.H., Peng, K.L., Jhang, H.C., Lin, C.H., Wu, S.Y., Chiang, C.M., Lee, S.C., Yu, W.C.Y. and Juan, L.J. (2012) The HPV E6 oncoprotein targets histone methyltransferases for modulating specific gene transcription. Oncogene 31, pp.2335–2349 . Available at: https://doi.org/10.1038/onc.2011.415
Institute of Medicine (US) Forum on Microbial Threats; Knobler SL, Mack A, Mahmoud A, et al., editors.(2005) The Threat of Pandemic Influenza: Are We Ready? Workshop Summary. Washington (DC): National Academies Press (US); 1, The Story of Influenza. Available from: https://www.ncbi.nlm.nih.gov/books/NBK22148/
Jablonka E. and Raz G. (2009): Transgenerational epigenetic inheritance: prevalence, mechanisms, and implications for the study of heredity and evolution. Q Rev Biol. 84(2):131-76. doi: 10.1086/598822. PMID: 19606595. Available online at: https://pubmed.ncbi.nlm.nih.gov/19606595/
Jackson R., Hellen C. and Pestova T. , (2010): The mechanism of eukaryotic translation initiation and principles of its regulation. Nat Rev Mol Cell Biol 11, 113–127 Available online at: https://doi.org/10.1038/nrm2838
Jaenisch R., and Bird A., (2003). Epigenetic regulation of gene expression: how the genome integrates intrinsic and environmental signals. Nature Genetics, 33(3), 245-254. Available online at: https://www.nature.com/articles/ng1089z
Kadayifci, F.Z., Zheng, S. and Pan, Y.-X. (2018). Molecular Mechanisms Underlying the Link between Diet and DNA Methylation. International Journal of Molecular Sciences, [online] 19(12). doi: https://doi.org/10.3390/ijms19124055
Kanwal M, Ding XJ, Cao Y. Familial risk for lung cancer. Oncol Lett. 2017 Feb;13(2):535-542. doi: 10.3892/ol.2016.5518. Epub 2016 Dec 20. PMID: 28356926; PMCID: PMC5351216.
Katherine A Aracena, Yen-Lung Lin, Kaixuan Luo, Alain Pacis, Saideep Gona, Zepeng Mu, Vania Yotova, Renata Sindeaux, Albena Pramatarova, Marie-Michelle Simon, Xun Chen, Cristian Groza, David Lougheed, Romain Gregoire, David Brownlee, Yang Li, Xin He, David Bujold, Tomi Pastinen, Guillaume Bourque, Luis B Barreiro.(2022) Epigenetic variation impacts ancestry-associated differences in the transcriptional response to influenza infection. bioRxiv 2022.05.10.491413. Available at: https://doi.org/10.1101/2022.05.10.491413
Keshavarz, M., Sabbaghi, A., Koushki, K., Miri, S.M., Sarshari, B., Vahdat, K. and Ghaemi, A. (2021). Epigenetic reprogramming mechanisms of immunity during influenza A virus infection. Microbes and Infection, 23(8), p.104831. Available online at: https://doi.org/10.1016/j.micinf.2021.104831.
Khan P, Siddiqui JA, Maurya SK, Lakshmanan I, Jain M, Ganti AK, Salgia R, Batra SK, Nasser MW. Epigenetic landscape of small cell lung cancer: small image of a giant recalcitrant disease. Semin Cancer Biol. 2022 Aug;83:57-76. doi: 10.1016/j.semcancer.2020.11.006. Epub 2020 Nov 18. PMID: 33220460; PMCID: PMC8218609.
Kim, K., Ryu, T.Y., Jung, E., Han, T.-S., Lee, J., Kim, S.-K., Roh, Y.N., Lee, M.-S., Jung, C.-R., Lim, J.H., Hamamoto, R., Lee, H.W., Hur, K., Son, M.-Y., Kim, D.-S. and Cho, H.-S. (2023). Epigenetic regulation of SMAD3 by histone methyltransferase SMYD2 promotes lung cancer metastasis. Experimental & Molecular Medicine, [online] pp.1–13. doi: https://doi.org/10.1038/s12276-023-00987-1
Kosiol C., Vinař T., da Fonseca R. R., Hubisz M J, Carlos D. Bustamante, Rasmus Nielsen, Adam Siepel (2008): Patterns of Positive Selection in Six Mammalian Genomes, Plos Genetics, 4(8), e1000144. Available online at: https://doi.org/10.1371/journal.pgen.1000144
Kronholm I. and Collins S., (2016): Epigenetic mutations can both help and hinder adaptive evolution. Molecular Ecology, 25(8), pp.1856-1868. Available online at: https://doi.org/10.1111/mec.13296
Lacal I., and Ventura R., (2018): Epigenetic Inheritance: Concepts, Mechanisms and Perspectives, Frontiers Molecular Neuroscience Available online at: https://www.frontiersin.org/journals/molecular-neuroscience/articles/10.3389/fnmol.2018.00292/full
Lai, Y, He, Z, Zhang, A, Yan, Z, Zhang, X, Hu, S, Wang, N and He, H (2020). Tip60 and p300 function antagonistically in the epigenetic regulation of HPV18 E6/E7 genes in cervical cancer HeLa cells. Genes Genom, 42, pp. 691–698. Available at: https://doi.org/10.1007/s13258-020-00938-4
Lakshminarasimhan R, Liang G. The Role of DNA Methylation in Cancer. Adv Exp Med Biol. 2016;945:151-172. doi: 10.1007/978-3-319-43624-1_7. PMID: 27826838; PMCID: PMC7409375.
Laland K.N., Uller T., Feldman M.W., Sterelny K., Müller G.B., Moczek A., Jablonka E., and Odling-Smee J. (2015): The extended evolutionary synthesis: its structure, assumptions and predictions. Proceedings of the Royal Society B: Biological Sciences, 282(1813), p.20151019. Available online at: https://doi.org/10.1098/rspb.2015.1019 [Accessed 19 July 2024].
Langevin, S.M., Kratzke, R.A. and Kelsey, K.T. (2015). Epigenetics of Lung Cancer. Translational research : the journal of laboratory and clinical medicine, [online] 165(1), pp.74–90. doi: https://doi.org/10.1016/j.trsl.2014.03.001
Lesch BJ, Tothova Z, Morgan EA, Liao Z, Bronson RT, Ebert BL, Page DC. Intergenerational epigenetic inheritance of cancer susceptibility in mammals. Elife. 2019 Apr 9;8:e39380. doi: 10.7554/eLife.39380. PMID: 30963999; PMCID: PMC6456297.
Lind M. I., and Spagopoulou F. (2018): Evolutionary consequences of epigenetic inheritance, Heredity121, 205–209. Available online at: https://doi.org/10.1038/s41437-018-0113-y
Liu, H, Ma, H, Li, Y and Zhao, H (2023). Advances in epigenetic modifications and cervical cancer research. Available at: https://www.sciencedirect.com/science/article/pii/S0304419X23000434
Liu, S., Liu, L., Xu, G., Cao, Z., Wang, Q., Li, S., Peng, N., Yin, J., Yu, H., Li, M., Xia, Z., Zhou, L., Lin, Y., Wang, X., Li, Q., Zhu, C., Yang, X., Wang, J., She, Y. and Lu, M. (2019). Epigenetic Modification Is Regulated by the Interaction of Influenza A Virus Nonstructural Protein 1 with the De Novo DNA Methyltransferase DNMT3B and Subsequent Transport to the Cytoplasm for K48-Linked Polyubiquitination. Journal of Virology, 93(7), pp.e01587-18. Available at: https://doi.org/10.1128/JVI.01587-18.
Liu, X., Jiao, B. and Shen, L. (2018). The Epigenetics of Alzheimer’s Disease: Factors and Therapeutic Implications. Frontiers in Genetics, [online] 9. Available at: https://www.frontiersin.org/journals/genetics/articles/10.3389/fgene.2018.00579/full [Accessed 19 Jul. 2024].
Lu Y., Chan YT., Tan HY. et al., (2020): Epigenetic regulation in human cancer: the potential role of epi-drug in cancer therapy. Mol Cancer 19, 79 Available online at: https://doi.org/10.1186/s12943-020-01197-3
Mac, Michelle and Moody, C.A (2020). Epigenetic Regulation of the Human Papillomavirus Life Cycle. Pathogens, 9(6), pp. 483. Available at: https://www.mdpi.com/2076-0817/9/6/483
Macartney E. L., Crean A. J., and Bonduriansky R. (2018): Epigenetic paternal effects as costly, condition-dependent traits. Heredity, 121(3), 248–256. Available online at: https://doi.org/10.1038/s41437-018-0096-8
MacLennan S. A., and Marra M. A., (2023): Oncogenic Viruses and the Epigenome: How Viruses Hijack Epigenetic Mechanisms to Drive Cancer, International Journal of Molecular Sciences 24, no. 11: 9543. Available online at: https://doi.org/10.3390/ijms24119543
Madia Lozupone, Dibello, V., Sardone, R., Castellana, F., Zupo, R., Lampignano, L., Bortone, I., Daniele, A., Bellomo, A., Solfrizzi, V. and Panza, F. (2023). The Impact of Apolipoprotein E (APOE) Epigenetics on Aging and Sporadic Alzheimer’s Disease. Biology, 12(12), pp.1529–1529. doi: https://doi.org/10.3390/biology12121529
Madlung A. and Comai L. (2004): The Effect of Stress on Genome Regulation and Structure, Annals of Botany, Vol. 94, Issue 4, pp481–495, Available online at: https://academic.oup.com/aob/article/94/4/481/189540?login=false
Marcos-Villar L, Díaz-Colunga J, Sandoval J, Zamarreño N, Landeras-Bueno S, Esteller M, Falcón A, Nieto A.(2018) Epigenetic control of influenza virus: role of H3K79 methylation in interferon-induced antiviral response. Sci Rep.19;8(1):1230.Available online at: https://pubmed.ncbi.nlm.nih.gov/29352168/
Martienssen R. and Slotkin R K. (2007): Transposable elements and the epigenetic regulation of the genome, Nature Reviews Genetics Available online at: https://www.nature.com/articles/nrg2072
Mboma A., (2020): Has Transgenerational Epigenetics Vindicated Jean-Bapstsiste Lamarck?, Epigenetics Lamarckian Evolution, Available online at: https://medium.com/an-idea/epigenetics-and-lamarckian-evolution-edc22c79a91c
Menachery, V.D., Schäfer, A., Burnum-Johnson, K.E., Mitchell, H.D., Eisfeld, A.J., Walters, K., Nicora, C.D., Purvine, S.O., Casey, C., Monroe, M.E., Weitz, K.K., Stratton, K.L., Webb-Robertson, B.-J.M., Gralinski, L.E., Metz, T.O., Smith, R.D., Waters, K.M., Sims, A.C., Kawaoka, Y. and Baric, R.S. (2018). MERS-CoV and H5N1 influenza virus antagonize antigen presentation by altering the epigenetic landscape. Proceedings of the National Academy of Sciences of the United States of America, 115(5). Available at: https://doi.org/10.1073/pnas.1706928115 .
Molaro A., Hodges E., Fang F., Song Q., McCombie W.R., Hannon G.J., and Smith A.D. (2011): Sperm methylation profiles reveal features of epigenetic inheritance and evolution in primates. Cell, 146(6), pp.1029-1041. Available online at: https://doi.org/10.1016/j.cell.2011.08.016
Mounger J., Ainouche M. L., Bossdorf O., Armand Cavé-Radet, Li B., Parepa M., Salmon A., Yang J., Richards C. L (2021): Epigenetics and the success of invasive species, Philos Trans R Soc Lond B Biol Sci. 2021 Jun 7;376(1826):20200117. doi: 10.1098/rstb.2020.0117. PMID: 33866809; PMCID: PMC8059582. Available online at: https://pubmed.ncbi.nlm.nih.gov/33866809/ and https://royalsocietypublishing.org/doi/10.1098/rstb.2020.0117
Nicolia, V. (2015). Environment, epigenetics and neurodegeneration: Focus on nutrition in Alzheimer’s disease. Experimental Gerontology, [online] 68, pp.8–12. Available at: https://www.sciencedirect.com/science/article/abs/pii/S0531556514002812 [Accessed 19 Jul. 2023].
Nogueira, M.O, Hošek, T, Calçada, E.O, Castiglia, F, Massimi, P, Banks, L, Felli, I.C and Pierattelli, P (2017).Monitoring HPV-16 E7 phosphorylation events . pp. 70-75. Available at: https://doi.org/10.1016/j.virol.2016.12.030
Penner-Goeke S., and Binder E. B., (2024): Epigenetics: Linking environmental factors and gene regulation, eLife 13:e96710.Available online at: https://elifesciences.org/articles/96710
Porter, S.S., Liddle, J.C., Browne, K., Pastrana, D.V., Garcia, B.A., Buck, C.B., Weitzman, M.D. and McBride, A. A (2021). Histone Modifications in Papillomavirus Virion Minichromosomes. mBio, 12(1). Available at: doi: 10.1128/mBio.03274-20
Ramazi, S., Daddzadi, M., Sahafnejad, Z. and Allahverdi, A. (2023). Epigenetic regulation in lung cancer. MedComm, [online] 4(6), p.e401. doi: https://doi.org/10.1002/mco2.401
Rastogi A., Lin X., Lombard B., Loew D., Tirichine L. (2015): Probing the evolutionary history of epigenetic mechanisms: what can we learn from marine diatoms, Aims Genetics vol.2 issue3, pp173-191, Available online at: https://doi.org/10.3934/genet.2015.3.173
Richards E. J. (2006): Inherited epigenetic variation—revisiting soft inheritance. Nature Reviews Genetics, 7(5), 395-401. Available online at: https://www.nature.com/articles/nrg1834
Richards, S., Aziz, N., Bale, S., Bick, D., Das, S., Gastier-Foster, J., Grody, W.W., Hegde, M., Lyon, E., Spector, E., Voelkerding, K. and Rehm, H.L., (2015): Standards and guidelines for the interpretation of sequence variants: a joint consensus recommendation of the American College of Medical Genetics and Genomics and the Association for Molecular Pathology. Genetics in Medicine, 17(5), pp.405-424. Available at: https://doi.org/10.1038/gim.2015.30
Rodger E.J., Chatterjee A., 2017. The epigenomic basis of common diseases. Clinical Epigenetics 9, 5 . Available online at: https://doi.org/10.1186/s13148-017-0313-y
Roy A., and Ghosh A., (2024): Epigenetic Restriction Factors (eRFs) in Virus Infection, Viruses16, no. 2: 183. Available online at: https://doi.org/10.3390/v16020183
Sana J., Faltejskova P., Svoboda M., and Slaby O. (2012) Novel classes of non-coding RNAs and cancer. J Transl Med10, 103. Available online at: https://doi.org/10.1186/1479-5876-10-103
Sezgin, Z. and Dincer, Y. (2014). Alzheimer’s disease and epigenetic diet. Neurochemistry International, [online] 78(78), pp.105–116. doi: https://doi.org/10.1016/j.neuint.2014.09.012
Sharma, S., Kelly, T.K. and Jones, P.A. (2009). Epigenetics in cancer. Carcinogenesis, [online] 31(1), pp.27–36. doi: https://doi.org/10.1093/carcin/bgp220
Slotkin R. K., and Martienssen, R. (2007): Transposable elements and the epigenetic regulation of the genome. Nature Reviews Genetics, 8(4), 272-285. Available online at: https://www.nature.com/scitable/content/transposable-elements-and-the-epigenetic-regulation-of-26513/
Sulewska, A.; Pilz, L.; Manegold, C.; Ramlau, R.; Charkiewicz, R.; Niklinski, J. A Systematic Review of Progress toward Unlocking the Power of Epigenetics in NSCLC: Latest Updates and Perspectives. Cells 2023, 12, 905. https://doi.org/10.3390/cells12060905
Tsunaka, Y., Kajimura, N., Tate, S., Morikawa, K. Protein Data Bank (PDB), 2005: Crystal structure of human nucleosome core particle, File entry name: 2CV5. Available online at: https://www.rcsb.org/structure/2cv5
Ulitsky I. (2016): Evolution to the rescue: using comparative genomics to understand long non-coding RNAs, Nature Reviews Genetics. Available online at: https://www.nature.com/articles/nrg.2016.85
Valente A., Vieira L., Silva M.J., and Ventura C., (2023): The Effect of Nanomaterials on DNA Methylation: A Review. Nanomaterials , 13, 1880. Available online at: https://doi.org/10.3390/nano13121880
Vecsey, C.G., Hawk, J.D., Lattal, K.M., Stein, J.M., Fabian, S.A., Attner, M.A., Cabrera, S.M., McDonough, C.B., Brindle, P.K., Abel, T. and Wood, M.A. (2007). Histone Deacetylase Inhibitors Enhance Memory and Synaptic Plasticity via CREB: CBP-Dependent Transcriptional Activation. Journal of Neuroscience, 27(23), pp.6128–6140. doi: https://doi.org/10.1523/jneurosci.0296-07.2007.
Veluchamy A., Rastogi A., Lin X., Lombard B., Murik O., Thomas Y., Dingli F., Rivarola M., Ott S., Liu X., Sun Y., Rabinowicz,P. D., McCarthy J., Allen A. E., Loew D., Bowler C., and Tirichine, L. (2015): An integrative analysis of post-translational histone modifications in the marine diatom Phaeodactylum tricornutum. Genome biology, 16(1), 102. Available online at: https://doi.org/10.1186/s13059-015-0671-8
Vergeer P., Wagemaker NC, Ouborg NJ.(2012): Evidence for an epigenetic role in inbreeding depression. Biol Lett. 23;8(5):798-801. doi: 10.1098/rsbl.2012.0494.. PMID: 22791708; PMCID: PMC3441007. Available online at: https://www.ncbi.nlm.nih.gov/pmc/articles/PMC3441007/ and https://doi.org/10.1098/rsbl.2012.0494
Waddington C. H. (2012). The epigenotype. 1942. International journal of epidemiology, 41(1), 10–13.Available online at: https://doi.org/10.1093/ije/dyr184
Weaver DF., (2023): Alzheimer’s disease as an innate autoimmune disease (AD2): A new molecular paradigm. Alzheimer’s Dement; 19: 1086–1098. Available online at: https://doi.org/10.1002/alz.12789
Ye, R, Wang, A, Bu, B, Luo, P, Deng, W, Zhang, X and Yin, S. (2023). Viral oncogenes, viruses, and cancer: a third-generation sequencing perspective on viral integration into the human genome. Available at: https://www.ncbi.nlm.nih.gov/pmc/articles/PMC10768168/
Yokoyama, A.S., Rutledge, J.C. and Medici, V. (2017). DNA methylation alterations in Alzheimer’s disease. Environmental Epigenetics, [online] 3(2). doi: https://doi.org/10.1093/eep/dvx008.
Zeng H., Shen E.H., Hohmann J.G., Oh S.W., Bernard A., Royall J.J., Glattfelder K.J., Sunkin S.M., Morris J.A., Guillozet-Bongaarts A.L., Smith K.A., Ebbert A.J., Swanson B., Kuan L., Page D.T., Overly C.C., Lein E.S., Hawrylycz M.J., Hof P.R., Hyde T.M., Kleinman J.E. and Jones A.R. (2012): Large-scale cellular-resolution gene profiling in human neocortex reveals species-specific molecular signatures. Cell, 149(2), pp.483-496. Available online at: https://doi.org/10.1016/j.cell.2012.02.052
Zeng J., Konopka G., Hunt B. G., Preuss T. M., Geschwind D., and Yi S. V. (2012). Divergent whole-genome methylation maps of human and chimpanzee brains reveal epigenetic basis of human regulatory evolution. American journal of human genetics, 91(3), 455–465. Available online at: https://doi.org/10.1016/j.ajhg.2012.07.024
Zhang Y. Y., Latzel V., Fischer M., and Bossdorf O. (2018). Understanding the evolutionary potential of epigenetic variation: a comparison of heritable phenotypic variation in epiRILs, RILs, and natural ecotypes of Arabidopsis thaliana. Heredity, 121(3), 257–265. Available online at: https://doi.org/10.1038/s41437-018-0095-9
Zygouras, I, Leventakou, D, Pouliakis, A, Panagiotou, S, Tsakogiannis, D, Konstantopoulos, G, Logotheti, E, Samaras, M, Kyriakopoulou, Z, Beloukas, A, Pateras, I.S., Delides, A, Psyrri, A, Panayiotides, I.G., Yiang, M, Kottaridi, C (2023). Human Papillomavirus 16 DNA Methylation Patterns and Investigation of Integration Status in Head and Neck Cancer Cases. 24(19), pp. 14593. Available at: https://doi.org/10.3390/ijms241914593