Supervised by: Yuhui Zhou, BA (Hons). Yuhui is a 5th year medical student at the University of Cambridge. She gained a First class degree in her intercalated year studying Pathology. She has an interest in Cancer & Immunology and has been awarded a Wellcome Trust Biomedical Vacation Scholarship to study host responses to infection.
Abstract
Friedreich’s Ataxia (FA) is a neurodegenerative disorder caused by a genetic mutation in the FXN gene located on chromosome 9q. This mutation decreases production of frataxin, a protein necessary for cellular respiration. Symptoms of Friedreich’s Ataxia include slurred speech, hearing loss, hypertrophic myopathy, and a gait. This research paper discusses some of the potential treatments for Friedreich’s Ataxia and discusses our current attempts at developing treatment plans for Friedreich’s Ataxia and some future plans.
1. Introduction
Friedreich’s ataxia (FA) is the most common autosomal recessive spinocerebellar ataxia. Ataxia is a term for a group of disorders that affect coordination, balance and speech (ataxia = a (lack of) + taxia (order). Ataxia usually results from damage to the part of the brain that controls muscle coordination (cerebellum) or its connections. There are three major groups of ataxia causes: acquired (caused by external factors including trauma, vitamin deficiencies, exposure to alcohol, drugs, toxins, thyroid problems, infections, or cancers), degenerative disease (multiple system atrophy and hereditary causes (occurs when a person has a damaged gene that is passed down from generation to generation).
In the 1860s, Nikolaus Friedreich, a professor of medicine at the University of Heidelberg, was the first to identify the disease but it remained difficult to distinguish from other spinocerebellar ataxias until the causative gene was determined in 1996 by Campuzano et al. The clinical criteria published by Harding in 1981 were the diagnostic tool of choice until 1996, when Campuzano et al. identified the causative alteration of the frataxin gene. This outcome paved the way for genetic testing. Even with the availability of genetic testing, the diagnosis of FA often relies on the exclusion of acute neurologic abnormalities and the presence of a slowly progressive clinical course.
1.2: FA Genetics
Some types of ataxias and some conditions that cause ataxia are hereditary. Different gene mutations cause different types of ataxias, most of which are progressive. All cause poor coordination, but each also has specific signs and symptoms. The hereditary ataxias can be inherited in an autosomal dominant, autosomal recessive, as part of a mitochondrial genetic syndrome. In both autosomal dominant and autosomal recessive disorders, the affected gene is present in an autosome (non-sex chromosome). In autosomal dominant conditions, one altered copy of the gene in a cell is sufficient for a person to be affected by the disease. In autosomal recessive condition, both recessive copies of the gene in a cell are needed for a person to be affected.
FA is a genetic disease and is caused by mutations on chromosome 9, in the gene coding for the frataxin protein (FXN).
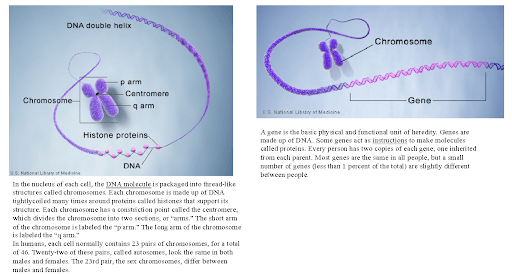
Figure 1: U.S. National Library of Medicine
FA is an autosomal recessive disease. Individuals who inherit two defective copies of the gene, one from each parent, will develop the disease. Both male and female children can inherit the disorder. Although rare (around 1 in 50000), FA is the most common form of hereditary ataxia. Symptoms usually first develop before the age of 25, although it can develop in people much older.
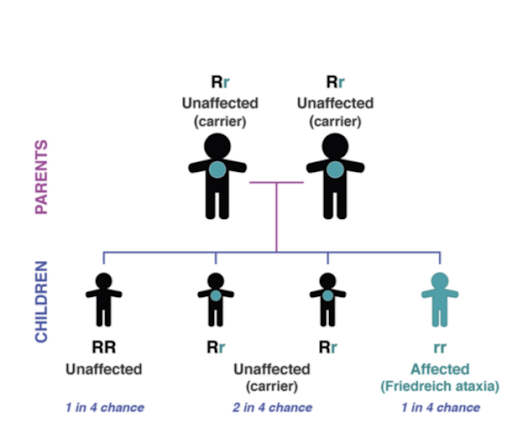
Figure 2: Autosomal Recessive Inheritance Pattern
Friedreich’s ataxia is caused by mutations on chromosome 9, in the gene coding for the frataxin protein (FXN). Frataxin is a mitochondrial protein involved in iron-sulfur cluster (Fe-S) cofactor biogenesis. However, the precise function of frataxin remains elusive.
Humans normally have 46 chromosomes in each cell, divided into 23 pairs. Two copies of chromosome 9, one copy inherited from each parent, form one of the pairs. Chromosome 9 is made up of about 141 million DNA building blocks (base pairs) and represents between 4.0% and 4.5% of the total DNA in cells. Chromosome 9 contains 800 to 900 genes that provide instructions for making proteins.
The single gene defect underlying FA was identified in 1996 as a large triplet expansion in intron 1 of the human FA gene (FXN) on chromosome 9q21.11.2. This expansion silences nuclear transcription of the mitochondrial targeted protein, frataxin (FXN), and causes decreased expression of this small protein. In the normal version of the gene, a triplet sequence of DNA (labeled guanine-adenine-adenine, or GAA) is repeated between 7 and 22 times. In the defective FXN gene, the GAA repeat occurs over and over again—hundreds, even up to a thousand times. The GAA repeat sequence greatly reduces the amount of frataxin produced by the cell. Earlier disease onset and severity of progression may be related to the number of GAA copies in the individual genetic code.
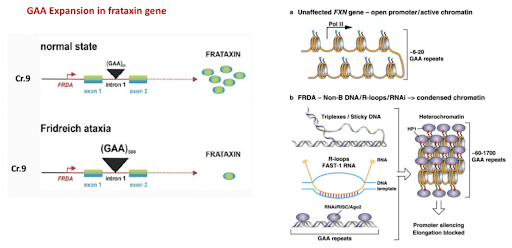
Figure 3: GAA Expansion in frataxin gene [source: ref 3]
1.3. FA pathophysiology
Frataxin is found in the energy-producing parts of the cell called mitochondria. Without a normal level of frataxin, mitochondria produce energy less effectively and have been hypothesized to have a build-up of toxic by-products leading to what is called “oxidative stress.” FXN gene expression and production of the frataxin are ubiquitous. However, the levels of mRNA and protein show tissue specificity that partially correlates with the main sites of disease. In humans, adults show the highest levels of expression in the heart and spinal cord, with intermediate levels observed in the cerebellum, liver, skeletal muscle, and pancreas, which are also the most affected sites in patients with FA.
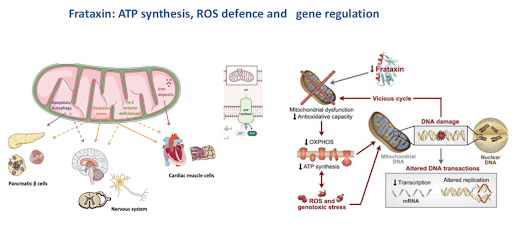
Figure 4 Frataxin: ATP synthesis, ROS defense and gene regulation [source: ref7]
All patients with FA have at least some frataxin, but tissues are not equally vulnerable. The concept of frataxin deficiency in FA, rather than the total absence of the protein, received a boost from a murine model. Total failure of frataxin biosynthesis causes embryonic lethality and it is incompatible with life.
The amount of remaining frataxin in FA patients ranges from 2% to 30%, depending on the extent of the silencing that is linked to the number of GAA-triplet repeats. The protein stores of frataxin in FA patients are 20%-25% of those seen in normal individuals, but we do not yet know how much protein is required for a normal phenotype.
The observation that all patients with FA have at least some frataxin has generated much interest in the possible reversal of the transcriptional block that is brought about by the overly long GAA trinucleotide repeats.
Abnormal frataxin production led to a marked decrease in enzymatic activities of mitochondrial and extra-mitochondrial iron-sulfur (Fe-S) cluster enzymes. FXN binds iron to participate in the formation of iron-sulfur (Fe-S) clusters in the mitochondrial matrix. Multiple key enzymatic systems within mitochondria depend on Fe-S clusters for their function, such as electron transport chain complexes I, II, and III, as well as aconitase in the Krebs cycle.
Fe-S clusters are protein cofactors providing catalytic activities to numerous enzymes with essential functions in ATP production, redox catalysis, protein and DNA synthesis, signaling as well as DNA maintenance. Any defect in their biosynthesis thus leads to metabolic defects affecting energy production and many other cellular functions. In addition to the Fe-S cluster biosynthesis defect, sensitivity to oxidative stress and decline in enzymatic activities of heme enzymes were also observed. Oxidative stress in FA patients is thought to result from the production of reactive oxygen species catalyzed by free iron accumulation in mitochondria (Fenton reaction) and from impaired oxidative stress signaling allowing reactive oxygen species to accumulate. In addition, mitochondrial FXN is essential for the generation of extra-mitochondrial Fe-S clusters that are used in cytosolic and nuclear locations.
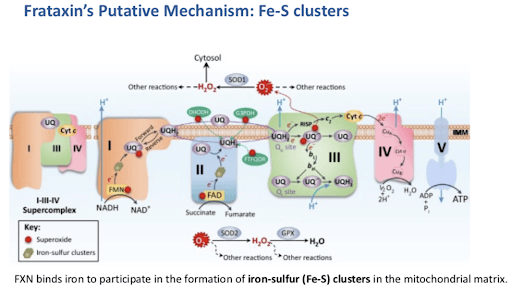
Figure 5: Frataxin’s Putative Mechanisms: Fe-S clusters [source; ref 8]
In this review, viral and non-viral vectors that have been used in glioblastoma gene therapy are discussed. Additionally, the differences, advantages and disadvantages of the two method.
1.4: FA Clinical Presentation
Many symptoms of Friedreich’s ataxia are related to abnormally low levels of frataxin, a protein that helps to protect cells from “free radicals,” which are toxic. As free radicals accumulate within cells, and more and more cells are destroyed or altered, the long-term effects of Friedreich’s ataxia led to a thinner spinal cord, enlarged heart muscle, disturbances in speech and eye movement, and loss of the pancreas’s ability to regulate blood sugar. Ultimately, almost everyone with Friedreich’s ataxia is confined to a wheelchair, and a large percentage of people develop serious heart problems, including heart failure.
The clinical manifestations include ataxia of the limbs and trunk, dysarthria, diabetes mellitus, and cardiac diseases.
FA exhibits variable phenotypes, and distinct early-onset and late-onset groups may be classified based on the symptom onset before or after age 25, respectively. Late-onset FA is characterized by less severe cardiomyopathy and neurologic symptoms, while early-onset typically exhibits more rapid progression with higher morbidity and mortality.
a. Neurological involvement
In Friedreich’s ataxia, nerve fibers in the spinal cord and peripheral nerves degenerate, becoming thinner. Peripheral nerves carry information from the brain to the body and from the body back to the brain, such as a message that the feet are cold or a signal to the muscles to generate movement. The cerebellum, which coordinates balance and movement, also degenerates to a lesser extent. This damage results in awkward, unsteady movements and impaired sensory functions. Major changes occur in the spinal cord, peripheral nerves, and cerebellum. Neurodegeneration occurs first in the dorsal root ganglia (DRG), with loss of large sensory neurons, followed by degeneration of posterior columns, corticospinal tracts and spinocerebellar tracts of the spinal cord, and the dentate nucleus in the cerebellum.
The major sites of neurodegeneration in FA patients are the dorsal root ganglia, the dorsal roots of the spinal cord, and the dentate nucleus in the cerebellum. It is not known why these regions of the nervous system are particularly sensitive to frataxin deficiency. Progressive atrophy of sensory and cerebellar pathways causes ataxia, dysarthria, gait instability, and profound sensory loss. The degeneration of corticospinal and pyramidal tracts leads to muscle weakness and extensor plantar responses.
A recent 5-year longitudinal study of more than 800 subjects with FA showed that the repeat size triplet expansion in first intron of the Friedreich ataxia gene (GAA) was the major determinant of neurologic progression in FA, and that a younger age at diagnosis also predicted faster progression.
b. Cardiac Involvement
60% of patients develop a hypertrophic cardiomyopathy that is associated with mitochondrial proliferation within the cardiomyocyte. This cardiomyopathy is unlike the sarcomere hypertrophic cardiomyopathies in that the hypertrophy is associated with massive mitochondrial proliferation within the cardiomyocyte rather than contractile protein overexpression. In FA cardiomyopathy, the mitochondria are abnormal in shape, size, and function, and animal studies have shown that the cardiomyocytes undergo chronic apoptosis. Because mitochondrial expansion within the cardiomyocyte is the predominant reason for cardiac hypertrophy in FA and most likely the cause of cardiomyocyte death, it is important to better understand the signaling between nucleus and mitochondria that results in this expansion.
Hypertrophic cardiomyopathy is associated with atrial arrhythmias, apoptosis, and fibrosis over time, and patients often develop heart failure leading to premature death.
Heart failure can develop in these patients with marked hypertrophy and may be associated with preserved ejection fraction. In later stages of FA heart disease, the ventricles may dilate and ejection fraction falls.
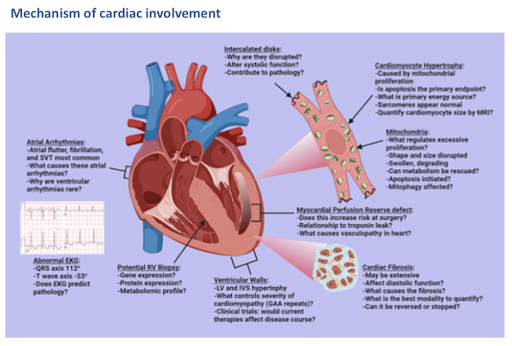
Figure 6. Mechanism of cardiac involvement [source: ref17]
Histopathology studies in FA Cardiomyopathy show:
Mitochondria within the cardiomyocytes appear abnormal with a wide variation in size, loss of cristae, enlargement, and electron dense deposits. Cardiomyocyte hypertrophy is associated with extensive mitochondrial proliferation and an increased prevalence of cardiomyocyte apoptosis. There is iron deposition within the heart.
The hypertrophic cardiomyopathy and death in FA do have a moderate association with the GAA triplet expansion length, and this correlation is not high enough to allow prediction of outcome. This is totally distinct to neurological involvement. Phenotypic response of the heart has also been noted to be highly variable between individuals with similarly sized GAA expansions, even between family members with the same expansion. There is significant disparity between the predictable advancement of the neurodegeneration versus the less predictable phenotype of cardiac involvement and progression.
1.5 Diagnosis
The diagnosis of Friedreich’s ataxia requires a careful clinical examination, which includes a medical history and a thorough physical exam. Among the tests performed are:
- Test of balance, joint sensation (proprioception), absence of reflexes, and signs of neurological problems
- Electromyogram (EMG), which measures the electrical activity of muscle cells
- Nerve conduction studies, which measure the speed with which nerves transmit impulses
- Electrocardiogram (ECG), which gives a graphic presentation of the electrical activity or beat pattern of the heart
- Echocardiogram, which records the position and motion of the heart muscle
- Blood tests to check for elevated glucose levels and vitamin E levels
- Magnetic resonance imaging (MRI) or computed tomography (CT) scans, tests which provide brain and spinal cord images that are useful for ruling out other neurological conditions
- Genetic testing involves taking a sample of blood and testing the DNA in it for any genetic mutation known to cause ataxia. Currently, tests can detect the mutations responsible for Friedreich’s ataxia
A recent study shows that even after the introduction of genetic testing, there is still a significant delay in clinical diagnosis of FA. The interval between the moment of symptom awareness and definite diagnosis, misdiagnosis may lead to poor disease management and counseling, over testing and inappropriate interventions, and/or delay to a causal therapy.
1.6 Correlations genotype/phenotype
This is less clear for the non-neurological symptoms (diabetes, cardiomyopathy, scoliosis). Intron 1 of the FXN gene typically contains up to about 30 GAA expansions. The pathological late onset starts at about 40–60 GAA on the shorter allele with a mean value of about 300 GAA. As the number of GAA increases, the onset of the disease occurs earlier, with an average value of approximately 600 GAA and up to 1,700 characterizing early onsets. This suggests a direct link between the level of remaining frataxin and the severity of the neurological features (onset and state of health). Expansion size has been shown to be associated with the severity of sensory neuropathy. Koeppel et al. recently reported that patients with late onset and long survival have significantly shorter GAA trinucleotide repeat expansions on both alleles.
Other two major complications of the disease are cardiomyopathy and diabetes. Cardiomyopathy frequently arises in patients with large expansions in the smaller allele and is independent of the duration of the disease.
Diabetes does not appear to be associated with either the number of GAA repeats or the duration of disease, but develops during the late stages of disease.
1.7 Gaps in Knowledge
Among the major knowledge gaps that remain to be solved for a better understanding of FA are:
- It is unknown what minimal level of FXN is needed to restore normal function in a cardiomyocyte and neuronal cells
- It is not known how FXN coordinates biogenesis of Fe-S clusters, or whether Fe-S cluster deficiency can be mitigated within the cell and mitochondria by alternative approaches
- It is also unknown and controversial whether FXN has functions in other locations within the cell, such as the nucleus or cytosol
- Because mitochondrial expansion within the cardiomyocyte likely contributes to cardiomyocyte death, it is important to better understand the signaling between nucleus and mitochondria that results in this expansion
- It is unknown whether FXN has a biochemical activity that can be directly measured
1.8 Understanding FTX Deficiency and Targets to Therapies
Targets for therapy are based on main phenotypes of frataxin deficiency, including antioxidant defense (idebenone, CoQ10 plus vitamin E), improvement of respiration (idebenone, CoQ10 plus vitamin E), reduction of the mitochondrial iron pools (deferiprone), and increase in frataxin protein (erythropoietin EPO, pioglitazone).
A major goal is the finding of chemical drugs that alleviate the Fe-S cluster deficiency.
Recent strategies target the GAA-expansion triplet repeat (sticky DNA structure and heterochromatin) and intend to increase the FXN gene expression (HDAC inhibitors, polyamides).
Other therapeutic strategies focus on replacing the mutated gene by gene therapy.
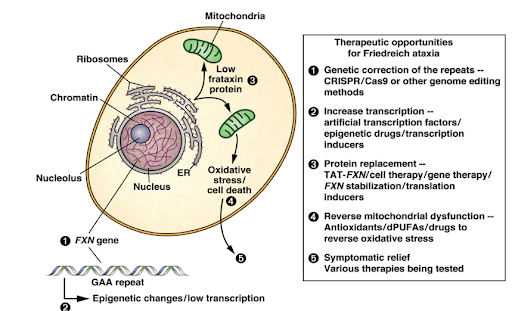
Figure included in Ref 3
2. Potential Treatments
Because of how rare FA is (affecting 1 in 50,000 people worldwide), very little research has been conducted to find treatments for this disease relative to other major illnesses such as cancer. Currently, medical treatments focus on pharmaceutical therapies for FA such as CTI-1601 and Coenzyme Q10 which offer promising results. Gene Therapy—reconstruction of mutated genes to produce a desired therapeutic effect (medlineplus.gov)–is an alternative option and offers similar, promising results.
2.1. Pharmaceutical Therapeutics
2.1.1: CTI-1601
Discovered by R. Mark Payne, MD, at the Indiana University School of Medicine, CTI-1601 is a recombinant fusion protein and trans-activator transcription (TAT) factor that transports synthetic frataxin directly into the mitochondria to make up for the lack of frataxin naturally produced by FA patients.
So far, CTI-1601 has only been tested using mice models but has proven to increase the average lifespan by 53% as well as improving heart function by increasing the heart rate and improving diastolic function Larimar Therapeutics, Inc., a biotechnology company focused on developing cures for rare complex diseases, states that CTTI-1601 has “been granted Rare Pediatric Disease designation, Fast Track designation and Orphan Drug designation by the U.S. Food and Drug Administration (FDA), Orphan Drug Designation by the European Commission, and PRIME designation by the European Medicines Agency.”
In May 2021, Larimar reported positive topline data from its Phase 1 FA program after completing a SAD (single ascending dose) trial in December 2020 and a MAD (multiple ascending dose) in March 2021. Data from these trials prove that daily subcutaneous injections of CTI-1601 for 13 consecutive days resulted in dose-dependent increases in frataxin levels compared to placebo. This occurred in all evaluated tissues (buccal cells, skin, and platelets). In buccal cells, frataxin levels following daily 50mg and 100mg subcutaneous injections of CTI-1601 were close to frataxin levels produced by heterozygous carriers of the mutated FXN gene.
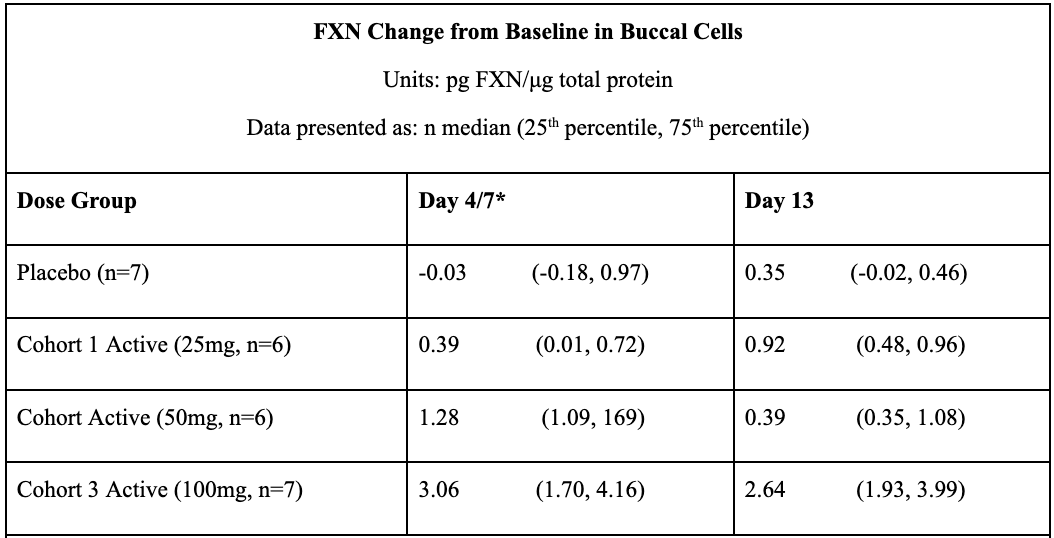
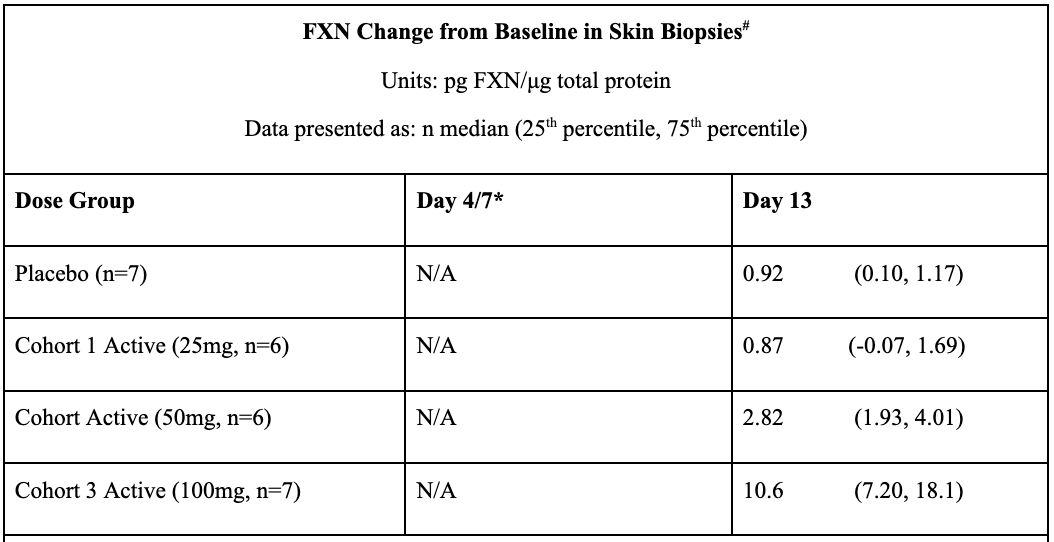
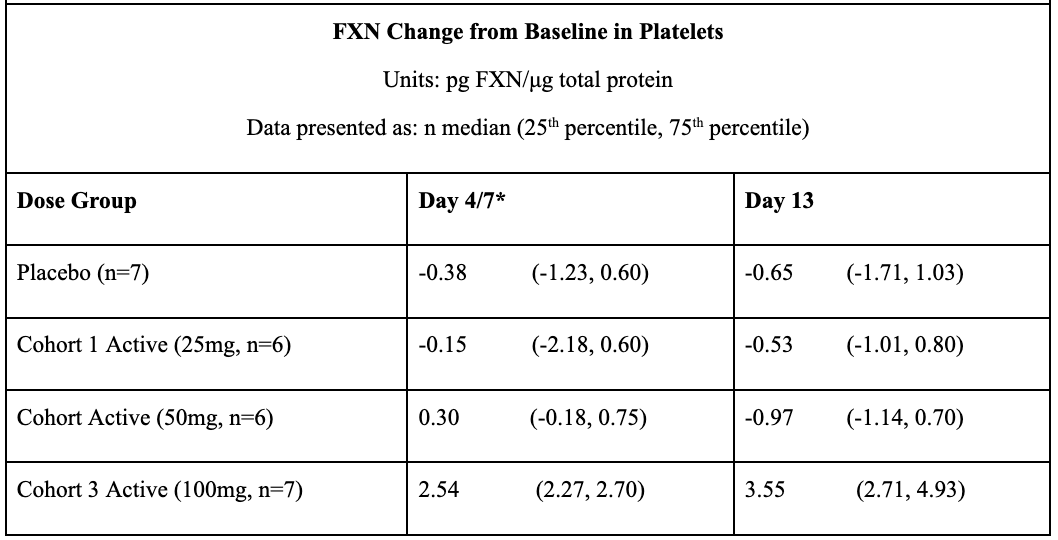
*FXN levels from Day 4 and 13 measurements are shown for data derived from Cohort 1. FXN levels from Day 7 and 13 measurements are shown for data derived from Cohorts 2 and 3. Sample collection days varied in each cohort per the clinical trial protocol.
#Skin biopsies were not collected on Day 4 or Day 7 per the clinical trial protocol. Skin biopsies were optional per the protocol and were collected in 5 of the 7 patients in Cohort 3.
Although there were no adverse effects in the SAD or MAD trials, on February 14, 2022, Larimar Therapeutics announced that the FDA had decided to maintain its clinical hold on CTI-1601 until additional data is provided to resolve the hold. The company was placed on hold following the company’s announcement to the FDA of the death of several non-human primates during a 26-week toxicology study.
2.1.2: Coenzyme Q10 (CoQ10)
Coenzyme Q10 (CoQ10) is an antioxidant agent your body naturally produces to use for growth and maintenance. Unlike CTI-1601, CoQ10 improves mitochondrial and cellular bioenergetics by decreasing oxidative stress in the mitochondria rather than providing the mitochondria with frataxin itself. The oxidant/antioxidant imbalance formed by the lack of frataxin are suspected to be a cause for problems in energy metabolism and may induce cellular degeneration, a great risk for neurodegenerative diseases.
However, there are many disease-specific proteins that interact with the mitochondria. Potential treatments such as CoQ10 which target mitochondrial processes (energy metabolism, for instance) hold great value (Mitochondrial dysfunction and oxidative stress in neurodegenerative diseases). In fact, CoQ10 has aided the recovery of those who have had bypass and heart valve surgeries as well as improving the symptoms of congestive heart failure and hypertrophic cardiomyopathy (Treatment of hypertrophic cardiomyopathy with coenzyme Q10), a symptom of FA. An open-label study showed improvements in cardiac and skeletal muscle bioenergetics using 400 mg/day of CoQ10 and 2100 IU/day of vitamin E on 10 FA patients. Over three months, the maximum rate of ATP production in skeletal muscles had increased by 139% of the original values.
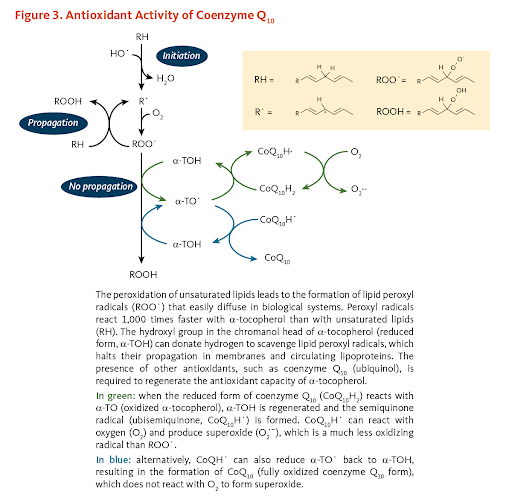
2.1: Pharmaceutical Therapeutics
2.2.1: Adeno-associated virus (AVV)
Unlike pharmaceutical therapeutics, gene therapy treats the underlying cause of a disease by correcting genetic mutations found in DNA. As we have previously established, Friedreich’s ataxia is caused by a GAA trinucleotide repeat in the FXN gene on chromosome 9q, the gene that codes for the protein frataxin which is instrumental in mitochondrial ATP production. Studies have shown that the more the GAA sequence is repeated, less frataxin is synthesized. An average human consists of 5-68 repeats while FA patients have 600-1500 repeats, explaining why they cannot synthesize frataxin efficiently. Essentially, the goal of gene therapy is to delete extra GAA repeats to make frataxin production more efficient. An increase in frataxin levels can significantly improve a patient’s health. Adeno-associated virus (AAV) vectors have proven to be a successful method to alter genetic material. In early 2014, researchers from Voyager Therapeutics used AAV to introduce a normal gene into the heart tissue of mouse models of FA to prove that viral vectors can prevent and rectify cardiac damage. Their hypothesis was proven correct as this treatment “restored the heart’s function and reversed heart enlargement in mice that had already developed heart failure, a symptom seen in people with FA”.
3. Future Prospects for FA
3.1: Current approaches – Introduction
Current approaches to FA study different treatments, some of which mainly focus on facing mitochondrial dysfunction by stimulating mitochondrial activity, increasing the levels of endogenous FXN with EPO (erythropoietin), epigenetic drugs, and immune modulators (IFNγ-1b). Nonetheless, previous treatments mentioned are palliative and are only studied in hopes of optimizing the quality of life and mitigating all existing sufferings of the patients.
Today, gene therapy remains to be the approach with the most promising results, where studies are focusing on viral vectors, such as the adeno-associated virus (AAV), in an attempt to deliver FXN to target cells. AAV is widely utilized in neurodegenerative diseases such as FA and ALS (Amyotrophic Lateral Sclerosis), whereas many studies are still testing the effectiveness of AAV serotypes (1-9) and rh10 using mouse models. In this section, we will discuss the effectiveness of AAV vectors and their success in replicating the pathogenesis of human FA in mouse models.
3.2: Mouse Models
An ongoing challenge in neurodegenerative diseases such as FA is the replication of the disease for test models. A difference between humans and mice is that human phenotypes allow survival under the circumstance of frataxin silencing, even at an embryonic state, whereas mice phenotypes deny this trait; for FA mice, the FXN protein is significantly crucial in embryonic development due to the finding that homozygous frataxin knockout (frataxin null) mice embryos were mortal to the mutation. The main approach made to avoid the fatality focused on in this review is the excision of exon 4 in selected genomes, which was essentially the conditional knockout of FXN in mice. Via the expression of the Cre-recombinase gene, researchers were able to conditionally knockout frataxin in selected cells and tissues.
3.3: AAV9 Coding for Frataxin
In a study to test for AAV(1-9) vectors, mice homozygous for the conditional frataxin allele (FA exon4 between 2 LoxP, [FRIDA L3/L3]) were crossed with heterozygous mice (HZM) for the excision of FDRA exon 4. The HZM also carried a Cre-transgene which granted researchers sophisticated dominion over the de/activation, timing, and location of gene expression, which was under the control of muscle creatine kinase (MCK) and a neuron-specific enolase (NSE) promoter respectively.
While the MCK promoter (tested models designated MCK-Cre) induced knockout of exon 4 in the heart and striated muscle, the NSE promoter (tested models designated NSE-Cre) administered exon 4 knockout in the heart, kidney, muscles, brain, and liver. As a result, the models were able to successfully reproduce pathophysiological and biochemical elements and traits of cardiac skeletal hypertrophy without the involvement of skeletal muscle.
Furthermore, the NSE-Cre mice exhibit large sensory neuron dysfunctions without alteration of the small sensory and motor neurons. The replication of features from said human diseases allows research and reliable testing of AAV vectors, while also overcoming the challenge of acquiring scarce human samples for study to a certain extent.
The investigation was brought out respective to the MCK-Cre and NSE-Cre models; the AAV Vector was delivered through intraperitoneal (IP) injection to reach target cells and tissues that have reduced or restricted expression of frataxin as per controlled by the Cre-transgene from embryogenesis. To evaluate the effectiveness of the vector, experimentation was also brought through respective dosages of the AAV9 vector (6 × 1011 v.p. → 6 × 109 v.p.), although it should be noted that for MCK-Cre mice, only the lowest and highest dosages were tested as the exorcism of frataxin in the heart lead to critical health issues, where intermediate doses provided similar-to-identical results and thus were insignificant.
3.4: Results
3.4.1: The confirmation of frataxin gene knockout was crucial to the reliability and availability of all regarded results
Using a Polymerase chain reaction (PCR) amplification, which is a conditional amplification of specifically targeted DNA or RNA by rapidly making millions to billions of copies of the selected sample, it was simple to verify the knockout of frataxin in the targeted cells and tissues of the model. In the MCK-Cre models, the delta allele (conditional frataxin allele) was only investigated in the muscle and the heart regarding the specialty of the MCK promoter, whereas for NSE-Cre models, the delta allele band was observed in all of the investigated tissues, which included the brain, the liver, the heart, the skeletal muscles, and the kidney.
3.4.2: NSE-Cre Models
Initially, all NSE-Cre mice were tested before AAV9-hFXN injection. At 5-8 weeks, they were usually stable and active without any major symptoms, however signs of kyphosis (convex curvature of thoracic and sacral regions) were evident and the mice walked on the tip of their toes, as displayed on the animal score below (Table 1, score 1). This is an indication of ectopic ossification, where the progression of this condition is monitored by the contracture of the limb joints. After complementary feeding, the models progressively exhibited ruffled fur, bent back, and bristly hairs; their general activity also significantly decreased. Their physical conditions were declining and rapidly degenerating, which resulted in a loss in body weight, and mobility/breathing/feeding difficulties by the end of their life (Table 1, score 3 and 4); at 35 ± 18 days (mean ± SEM), all models were sacrificed due to ethical regards.
An AAV vector coded for human frataxin (AAV9-hFXN) was delivered through IP injection to models at 5-9 days of age. With concern to body weight, a growth curve was carefully studied along NSE-Cre models that were treated and untreated with the AAV9-hFXN. With L3/L3 mice as an ideal illustration, the NSE-Cre mice growth curve was found to be significantly lower than that of the former. For NSE-Cre specimens that received the AAV9-hFXN treatment, the results of the treated models presented a significantly improved growth curve over the untreated models. Nonetheless, none of the tested models were able to reach the ideal standard of the L3/L3 models for both male and female specimens, with exception of only 3 male models receiving an injected dosage of 6 × 1011 v.p. (d1/10) seemed to have followed the curve of the L3/L3 standard; although treatments prove an effect on the NSE-Cre models, it was yet to improve mice growth to match healthy and ideal standards.
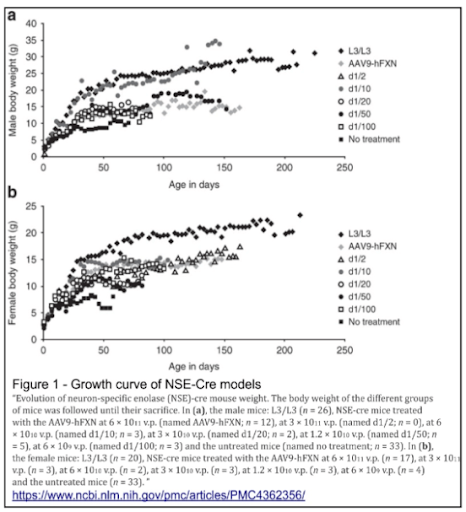
To test for the presence of human frataxin transgene, the study used a PCR for the human frataxin (post sacrifice), which allowed a more detailed analysis and study of the genome. The investigation was brought out in the heart, brain, kidney, muscles, and liver, where the transgene was detected in all areas (for 6 × 10^11 v.p. dosage). After dilutions 1/10 and 1/20 were tested, it was concluded that although the dilutions decreased the intensity of the human frataxin band in all organs, the strongest band always remained in the heart; this was also the case from results from collected RT-PCR samples.
It should be also noted that there is no presence whatsoever of the human frataxin transgene in L3/L3 mice, which have been injected with saline. However, for L3/L3 mice injected IP with the highest dosage of the AAV9-hFXN, similar results as the NSE-Cre models were given, and they were found to have strong band intensity of the human frataxin in all tissues. The presence of human frataxin was strong with the highest dose of virus (6 × 10^11 v.p. [n=9]) for all models tested, with lower dosages exhibiting lower band intensity and diminished expression levels, except for the heart where the intensity remained strong despite lower dosages.
In general, what does this all mean for the collected results? After conducting all investigations, estimation of the NSE-Cre model average health was assessed with a score from 0-4, where a score of 0 corresponds to having no symptoms and a score of 4 indicates that the state of health requires immediate sacrifice due to ethical concerns. All NSE-Cre models were evaluated and monitored after weaning (21 days) as the disease was found to have a great variability of progression and mortality, as mentioned above.
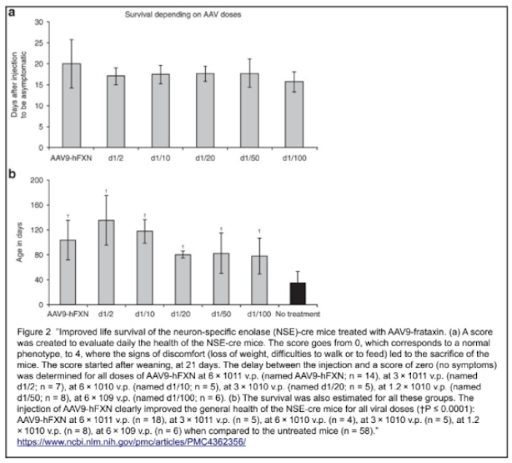
Although there were no evident differences in the heart weight for L3/L3, NSE-Cre and treated NSE-Cre models at the time of sacrifice, there was a significant difference in body weight, hence the weight of the heart is expressed as a percentage of the body weight in comparison. To summarize the percentage readings, the higher the index the worse the mouse health is as the body weight is diminished. For the L3/L3 mice, the reading was 0.57 ± 0.11. For NSE-Cre models, all dosages of treatment proved to be strongly effective; untreated models displayed a percentage of 1.91 ± 0.61 to 0.81 ± 0.23 with the AAV9-hFXN treatment 6 × 10^11 v.p..
All treated NSE-Cre models were found to be in largely different conditions from the untreated NSE-Cre models, however not significantly unsimilar to the condition of the L3/L3 models. This proves the effectiveness of AAV9-hFXN treatment of any dosage on NSE-Cre models with frataxin knockout, where results are promising to treat the human disease as treated models are progressively showing better health conditions to match with the L3/L3 model health standard.
3.4.3: MCK-Cre Models
MCK-Cre models exhibit a major health issue due to the excision of frataxin in the heart. NSE-Cre models exhibited a strong presence of human frataxin in the heart with just a minimum dose of 6 x 10^9 v.p. of AAV9-hFXN vector; in this study for MCK-Cre models, only the lowest and highest dosages are tested.
Unlike NSE-Cre models, the MCK-Cre models displayed the same body weight and growth curve as the L3/L3 models, whereas after IP administration of AAV9-hFXN at 6 × 1011 v.p. and 6 × 109 v.p., there was no significant difference evident to the growth curve of models without treatment.
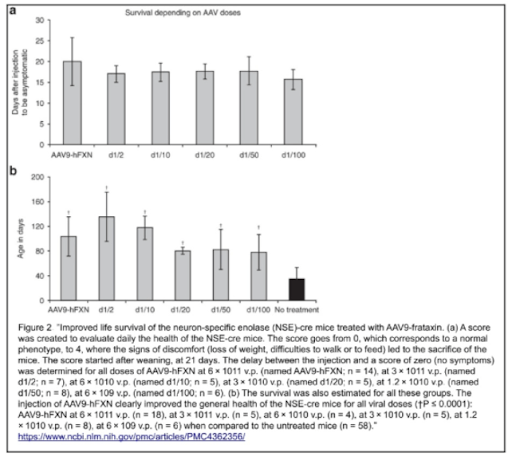
A PCR was used to detect the presence of the virus in genomic DNA, and using an RT-PCR to detect human frataxin after 6 x 10^9 v.p. treatment. While NSE-Cre models exhibited the strongest frataxin band in the heart, the MCK-Cre models had stronger bands in the heart, muscle, and liver. Both 6 x 10^9 v.p. and 6 x 10^11 v.p. dosages we tested for the MCK-Cre models and all organs were observed except for the brain, which had no presence of the protein at all. However, the frataxin concentration remained lower than that of the concentration of the virus.
Nonetheless, the AAV9-hFXN vector still proved to be a great success for MCK-Cre models. At a young age, MCK-Cre models and L3/L3 models displayed no significant differences in behavior, and their body weight and activity were all similar. However, the MCK-Cre mice’s health started to regress suddenly and decline rapidly, where they had difficulty breathing and walking and had to be sacrificed after 24-48 hours due to ethical reasons. MCK-Cre models with IP administration of the highest dosage of AAV-hFXN showed a great improvement in health; while untreated mice had a lifespan of 71 ± 20 days, models with 6 x 10^11 v.p. dosage of AAV had a lifespan of 194 ± 67 days. Both the lowest and highest dosage of the vector exhibited a great difference in health and lifespan for MCK-Cre mice compared to untreated models, however, the gap remains in the heart weight percentage to the body mass that of the L3/L3 control model due to evident cardiac hypertrophy.
From echocardiography results, the highest dosage of AAV9-hFXN reformed stroke volume, cardiac output, and the diastolic and systolic diameters in the MCK-Cre models. On a similar note, IP administration of the AAV9-hFXN was proven to not have any effect on the stroke output or the cardiac output of the NSE-Cre models but restored the diastolic and systolic parameters and function in the heart.
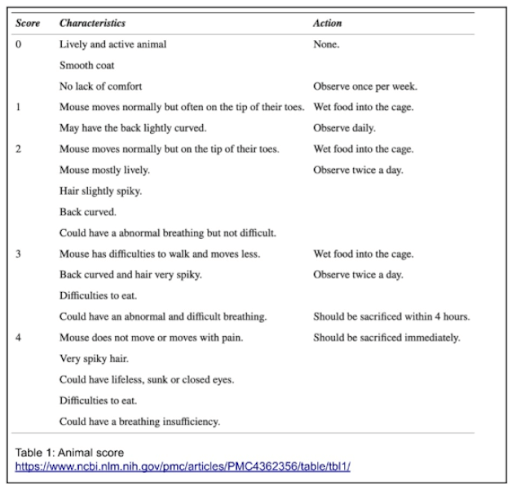
4. Conclusion
4.1: Current Attempts
In our opinion, we believe gene therapy is currently most effective in tackling Friedreich’s ataxia because, unlike pharmaceutical therapeutics, it has the potential to treat the root cause of the FA rather than just the symptoms. Although there is not much numerical data to prove this, new forms of gene therapy are forming to improve our results. Adeno-associated virus (AAV) vectors only treat targeted skeletal and muscular tissue, results of the FXN gene being mutated. Because genetic modification is a relatively new form of treatment and not much research has been conducted in this field, AAV vectors seem to be our only feasible option for genetic modification. However, new forms of genetic modification are emerging such as CRISPR-AAV, a combination of CRISPR Cas9 technology with AAV, to delete desired genes, such as the GAA repeat, something our current technology cannot do. With newly developed mouse models and more clinical trials for mitochondrial diseases, there is still a lot more to uncover about FA. However, the results for current studies have been promising thus far.
4.2: Future Prospects
MCK-Cre models have exorcized frataxin in the heart and muscles where the Cre-recombinase has been expressed for transgene control, while NSE-Cre models only have a complete knockout in the heart and partial knockout in all other organs. For the study of FA, MCK-Cre models have more cardiac-specific symptoms than the human disease FA, being the lack of frataxin in almost every organ. However, NSE-Cre models still proved to be useful in this study due to having severe symptoms at earlier onset stages, hence allowing clearer detection of symptoms before severe progression of the disease.
Today, studies are able to replicate more symptoms from human diseases, but the successes are limited; due to the difference in human and mice phenotypes, we are yet to discover the true reliability and effect of the treatment on the human disease, and although we have newfound success in conditional knockout mice, the results are not viable to address the issue in the dynamics of GAA expansion mutation, which is also a promising prospect.
Works Cited
- Almeida, Maria Joao. “Gene Therapy for Friedreich’s Ataxia.” Friedreich’s Ataxia News, 16 Aug. 2017, friedreichsataxianews.com/gene-therapy-for-friedreichs-ataxia/.
- Beal, Flint, and Micheal T. Lin. “Mitochondrial Dysfunction and Oxidative Stress in Neurodegenerative Diseases.” Nature, U.S. National Library of Medicine, 18 Oct. 2006, pubmed.ncbi.nlm.nih.gov/17051205/.
- Calabrese, Vittorio, et al. “Oxidative Stress, Mitochondrial Dysfunction and Cellular Stress Response in Friedreich’s Ataxia.” Journal of the Neurological Sciences, Elsevier, 17 May 2005, www.sciencedirect.com/science/article/abs/pii/S0022510X05000997.
- Campuzano, Victoria, et al. “Frataxin Is Reduced in Friedreich Ataxia Patients and Is Associated with Mitochondrial Membranes.” OUP Academic, Oxford University Press, 1 Oct. 1997, academic.oup.com/hmg/article/6/11/1771/684122?login=false.
- Campuzano, Victoria, et al. “Friedreich’s Ataxia: Autosomal Recessive Disease Caused … – Researchgate.” ResearchGate, Apr. 1996, www.researchgate.net/publication/224973869_Friedreich’s_Ataxia_Autosomal_Recessive_Disease_Caused_by_an_Intronic_GAA_Triplet_Repeat_Expansion.
- Coenzyme Q10, Oregon State University, lpi.oregonstate.edu/book/export/html/352.
- Collins, Abigail. “Clinical Neurogenetics Friedreich Ataxia.” Neurological Clinics, 2013, www.neurologic.theclinics.com/article/S0733-8619(13)00059-5/fulltext.
- Cossée, Mireille, et al. “Inactivation of the Friedreich Ataxia Mouse Gene Leads to Early Embryonic Lethality without Iron Accumulation.” OUP Academic, Oxford University Press, 1 May 2000, academic.oup.com/hmg/article/9/8/1219/604977?login=false.
- Cossée, Puccio M. “Inactivation of the Friedreich Ataxia Mouse Gene Leads to Early Embryonic Lethality without Iron Accumulation.” Europe PMC, 1 May 2000, europepmc.org/article/med/10767347.
- “CTI-1601.” Larimar Therapeutics, 2022, larimartx.com/our-programs/cti-1601/.
- Cynwyd, Bala. “Larimar Therapeutics Reports Second Quarter 2021 Operating and Financial Results.” Sec.gov, Larimar Therapeutics, 12 Aug. 2021, www.sec.gov/Archives/edgar/data/0001374690/000119312521244068/d202246dex991.htm.
- Delatycki, Martin B., et al. “Clinical Features of Friedreich Ataxia.” SAGE Journals, 29 June 2012, journals.sagepub.com/doi/abs/10.1177/0883073812448230.
- Filla, A, et al. “The Relationship between Trinucleotide (GAA) Repeat Length and Clinical Features in Friedreich Ataxia.” American Journal of Human Genetics, U.S. National Library of Medicine, Sept. 1996, www.ncbi.nlm.nih.gov/pmc/articles/PMC1914893/.
- Gottesfeld, Joel M. “Molecular Mechanisms and Therapeutics for the GAA·TTC Expansion Disease Friedreich Ataxia.” Neurotherapeutics : the Journal of the American Society for Experimental NeuroTherapeutics, Springer International Publishing, Oct. 2019, www.ncbi.nlm.nih.gov/pmc/articles/PMC6985418/.
- Grabczyk, Ed, and Karen Usdin. “GAA•TTC Triplet Repeat Expanded in Friedreich’s Ataxia Impedes Transcription Elongation by T7 RNA Polymerase in a Length and Supercoil Dependent Manner.” OUP Academic, Oxford University Press, 15 July 2000, academic.oup.com/nar/article/28/14/2815/2383765.
- Gérard , Catherine, et al. “An AAV9 Coding for Frataxin Clearly Improved the Symptoms and Prolonged the Life of Friedreich Ataxia Mouse Models.” Molecular Therapy. Methods & Clinical Development, U.S. National Library of Medicine, 8 Oct. 2014, pubmed.ncbi.nlm.nih.gov/26015982/.
- Gómez-Serrano, María, et al. “Mitoproteomics: Tackling Mitochondrial Dysfunction in Human Disease.” Oxidative Medicine and Cellular Longevity, Hindawi, 8 Nov. 2018, www.ncbi.nlm.nih.gov/pmc/articles/PMC6250043/.
- Gómez-Serrano, María, et al. “Mitoproteomics: Tackling Mitochondrial Dysfunction in Human Disease.” Oxidative Medicine and Cellular Longevity, Hindawi, 8 Nov. 2018, www.ncbi.nlm.nih.gov/pmc/articles/PMC6250043/.
- Hanson, Emily, et al. “Heart Disease in Friedreich’s Ataxia.” World Journal of Cardiology, Baishideng Publishing Group Inc, 26 Jan. 2019, www.ncbi.nlm.nih.gov/pmc/articles/PMC6354072/#:~:text=The%20cardiac%20diseases%20of%20FRDA,are%20the%20most%20common%20clues.
- Harding, A. E. “Friedreich’s Ataxia: A Clinical and Genetic Study of 90 Families with an Analysis of Early Diagnostic Criteria and Intrafamilial Clustering of Clinical Features.” OUP Academic, Oxford University Press, 1 Sept. 1981, academic.oup.com/brain/article-abstract/104/3/589/296804?redirectedFrom=fulltext&login=false.
- Indelicato, Elisabetta, et al. “Onset Features and Time to Diagnosis in Friedreich’s Ataxia.” BioMed Central, BioMed Central, 3 Aug. 2020, ojrd.biomedcentral.com/articles/10.1186/s13023-020-01475-9.
- Koeppen, Amulf H. “Friedreich’s Ataxia: Pathology, Pathogenesis, and Molecular Genetics.” Journal of the Neurological Sciences, 11 Feb. 2011, www.jns-journal.com/article/S0022-510X(11)00012-8/fulltext.
- Koeppen, Arnulf H., et al. “The Neuropathology of Late-Onset Friedreich’s Ataxia – the Cerebellum.” SpringerLink, Springer-Verlag, 4 Dec. 2010, link.springer.com/article/10.1007/s12311-010-0235-0.
- “Larimar Therapeutics Reports Positive Topline Phase 1 Clinical Trial …” Larimar Therapeutics, Larimar Therapeutics, 11 May 2021, investors.larimartx.com/node/10581/pdf.
- Lynch, David R, and Garrett Farmer. “Mitochondrial and Metabolic Dysfunction in Friedreich Ataxia: Update on Pathophysiological Relevance and Clinical Interventions.” Neuronal Signaling, Portland Press Ltd., 17 May 2021, www.ncbi.nlm.nih.gov/pmc/articles/PMC8132591/.
- Mayo Clinic Staff. “Coenzyme Q10.” Mayo Clinic, Mayo Foundation for Medical Education and Research, 10 Nov. 2020, www.mayoclinic.org/drugs-supplements-coenzyme-q10/art-20362602.
- “Mitochondrial Disease.” Children’s Hospital of Philadelphia, The Children’s Hospital of Philadelphia, 9 May 2014, www.chop.edu/conditions-diseases/mitochondrial-disease#:~:text=Currently%20there%20is%20no%20highly.
- “Mitochondrial Diseases: Causes, Symptoms, Diagnosis & Treatment.” Cleveland Clinic, Cleveland Clinic, 31 May 2018, my.clevelandclinic.org/health/diseases/15612-mitochondrial-diseases.
- Monfort, Beata, et al. “Recent Advances in the Elucidation of Frataxin Biochemical Function Open Novel Perspectives for the Treatment of Friedreich’s Ataxia.” Frontiers, Frontiers, 2 Mar. 2022, www.frontiersin.org/articles/10.3389/fnins.2022.838335/full.
- Montermini, Laura, et al. “Phenotypic Variability in Friedreich Ataxia: Role of the Associated GAA Triplet Repeat Expansion.” Wiley Online Library, 8 Oct. 2004, doi.org/10.1002/ana.410410518.
- Nelwan, Martin. “Friedreich Ataxia: Treatment with Genetic Approach.” SSRN, SSRN, 15 June 2017, papers.ssrn.com/sol3/papers.cfm?abstract_id=2986633.
- Ocana-Santero, Gabriel, et al. “Future Prospects of Gene Therapy for Friedreich’s Ataxia.” International Journal of Molecular Sciences, U.S. National Library of Medicine, 11 Feb. 2021, pubmed.ncbi.nlm.nih.gov/33670433/.
- Pallardo, Fedrico V., et al. Translational Research, 22 Aug. 2020, www.translationalres.com/article/S1931-5244(20)30204-8/fulltext.
- Pandolfo, Massimo, and Annalisa Pastore. “The Pathogenesis of Friedreich Ataxia and the Structure and Function of Frataxin – Journal of Neurology.” SpringerLink, Steinkopff-Verlag, link.springer.com/article/10.1007/s00415-009-1003-2.
- Payne, R. Mark. “Cardiovascular Research in Friedreich Ataxia: Unmet Needs and Opportunities.” JACC: Basic to Translational Science, Elsevier, 13 July 2022, www.sciencedirect.com/science/article/pii/S2452302X22001425.
- Selvadurai, Louisa P., et al. “Cerebral Abnormalities in Friedreich Ataxia: A Review.” Neuroscience & Biobehavioral Reviews, Pergamon, 18 Aug. 2017, www.sciencedirect.com/science/article/abs/pii/S0149763417301860.
- Simon, Delphine, et al. “Friedreich Ataxia Mouse Models with Progressive Cerebellar and Sensory Ataxia Reveal Autophagic Neurodegeneration in Dorsal Root Ganglia.” The Journal of Neuroscience : the Official Journal of the Society for Neuroscience, U.S. National Library of Medicine, 25 Feb. 2004, pubmed.ncbi.nlm.nih.gov/14985441/.
- Tai, Geneieve, et al. “Progress in the Treatment of Friedreich Ataxia.” Neurologia i Neurochirurgia Polska, U.S. National Library of Medicine, 2018, pubmed.ncbi.nlm.nih.gov/29499876/.
- “What Are Genome Editing and CRISPR-Cas9?: Medlineplus Genetics.” MedlinePlus, U.S. National Library of Medicine, medlineplus.gov/genetics/understanding/genomicresearch/genomeediting/.
- “What Is Gene Therapy?: Medlineplus Genetics.” MedlinePlus, U.S. National Library of Medicine, 28 Feb. 2022, medlineplus.gov/genetics/understanding/therapy/genetherapy/.